- Department of Radiation Oncology, Affiliated Jinling Hospital, Medical School of Nanjing University, Nanjing, China
Radiotherapy is one of the mainstays of cancer treatment. More than half of cancer patients receive radiation therapy. In addition to the well-known direct tumoricidal effect, radiotherapy has immunomodulatory properties. When combined with immunotherapy, radiotherapy, especially high-dose radiotherapy (HDRT), exert superior systemic effects on distal and unirradiated tumors, which is called abscopal effect. However, these effects are not always effective for cancer patients. Therefore, many studies have focused on exploring the optimized radiotherapy regimens to further enhance the antitumor immunity of HDRT and reduce its immunosuppressive effect. Several studies have shown that low-dose radiotherapy (LDRT) can effectively reprogram the tumor microenvironment, thereby potentially overcoming the immunosuppressive stroma induced by HDRT. However, bridging the gap between preclinical commitment and effective clinical delivery is challenging. In this review, we summarized the existing studies supporting the combined use of HDRT and LDRT to synergistically enhance antitumor immunity, and provided ideas for the individualized clinical application of multimodal radiotherapy (HDRT+LDRT) combined with immunotherapy.
Introduction
Radiotherapy has long been the cornerstone of cancer treatment for curative purposes as well as palliative relief of symptoms, thereby improving quality of life (1–4). In addition to inducing irreparable DNA damage with direct cytotoxic effects on cancer cells, there is growing evidence that radiotherapy can modulate the immune system, resulting in systemic antitumor immunity (5, 6). This systemic response is called abscopal effect, that is, radiation targeting one tumor lesion can induce in situ vaccination by killing tumor cells, and then lead to the regression of distant unirradiated tumors (7–9). Although radiotherapy alone rarely induces abscopal effects, the potential systemic antitumor ability provides a good basis for radiotherapy combined with immunotherapy.
Immunotherapy has been recognized as an effective oncologic therapy. In particular, immune checkpoint inhibitors (ICIs) have achieved surprising clinical efficacy in the treatment of advanced solid tumors (10–12). However, only a minority of patients with advanced cancers can experience persistent and stable benefit from ICIs alone (13). As a result, many clinical trials are exploring the synergistic effect of radiation therapy combined with immunotherapy to enhance antitumor immunity (14–18). The updated data of PACIFIC Trial demonstrated robust and sustained overall survival (OS) and durable progression free survival (PFS) benefit with durvalumab after chemoradiotherapy in patients with unresectable, stage III non-small-cell lung cancer (NSCLC) (19). These compelling clinical evidences provide a basis for further exploration of the best combination regimen.
The stereotactic body radiation therapy (SBRT, also known as stereotactic ablative radiotherapy [SABR]) is increasingly used to deliver highly targeted high doses with fewer fractions, because of the high rates of local tumor control with tolerable toxicity (20–22). In addition, high-dose radiotherapy (HDRT, such as SBRT) is more immunogenic than conventional radiotherapy. HDRT can mobilize innate and adaptive immunity against tumors (23–26). Therefore, scholars focused on the combination of HDRT with immunotherapy to enhance the antitumor immunity of patients. Several clinical studies have shown that SBRT combined with ICIs can significantly improve the response rates in metastatic tumors with well tolerated (27–29). Despite previous progression on anti-PD-1 therapy, SBRT has reinvigorated a systemic response (30). Nevertheless, in some cases, SBRT in conjunction with ICIs may not eliminate distant tumors, and benefit only a small fraction of patients (31, 32). HDRT can sometimes have inhibitory effects on antitumor immunity, such as recruiting immunosuppressive cells and increasing the secretion of immunoregulatory cytokines (33, 34). It is urgent to overcome the immune-suppressive barriers to increase the beneficiary population of immunotherapy.
Many studies have shown that low-dose radiotherapy (LDRT), i.e., lower than 2 Gy/fraction, can effectively reprogram the stroma from an immunosuppressive to immunostimulatory and synergize with immunotherapy (35–37). Interestingly, the mechanisms by which HDRT and LDRT regulate the antitumor immune system appear to be complementary (38). Therefore, the use of the multimodal radiotherapy regimen, such as HDRT and LDRT, can achieve optimal antitumor effects. We first proposed the concept of multimodal radiotherapy, that is, the combination of different radiotherapy modalities, such as SBRT combined with intensity modulated radiotherapy (IMRT), SBRT combined with LDRT, HDRT combined with LDRT, etc. Savage et al., compared a single-dose ablative fractionation of 24Gy with 22Gy followed by 4 fractions of 0.5Gy targeting the local tumor in C57BL/6 mice. They found that the addition of LDRT delayed local tumor progression and significantly improved survival. In addition, survival was significantly increased after whole-lung radiated by low dose (0.5Gyx4f), 12 days after completion of the primary tumor radiation (20Gyx3f) (39). Furthermore, some preclinical and clinical studies showed that the multimodal radiotherapy (HDRT and LDRT) combined with immunotherapy can enhance systemic anti-tumor immune responses (40–44). However, there are many problems about this novel combination therapy strategy. For example, the selection of immunotherapy agents, the sequence of multimodal radiotherapy combined with immunotherapy, the dose of radiation, the number of fractions, the site of high-dose irradiation, and the site of low-dose irradiation.
In this review, we discussed the optimal radiotherapy regimens for enhancing antitumor immunity. First, we investigated the modulation of radiation on the immune system, including immunoenhancing and immunosuppressive effects. Furthermore, we described the different mechanisms of HDRT and LDRT in immune regulation. Finally, we studied the rationale for combining multimodal radiotherapy (HDRT and LDRT) with immunotherapy to enhance antitumor immune responses.
The direct killing effect of radiation on tumor cells
Radiation therapy has been widely used to treat malignant tumors since the discovery of X-ray by Roentgen in 1895 (45). Approximately 60-70% of cancer patients require radiation therapy during treatment (7). In 1911, Regaud et al. proposed the concept of fractionated radiotherapy, in which a large doses can be divided into fractions over days or weeks (46). Nowadays, the conventional fractionated radiotherapy regimen is usually 1.8-2 Gy daily, 5 fractions/week. Hypofractionated radiotherapy refers to increasing the single irradiation dose >2 Gy, which has the advantage of shortening the treatment time span of patients and avoiding accelerated tumor proliferation after radiotherapy. Over the past few decades, the field of radiation has undergone tremendous technological innovations that can significantly reduce radiation damage to healthy tissues with modern radiotherapy techniques such as helical tomotherapy, IMRT, proton radiotherapy, SBRT and FLASH radiotherapy (22, 47–52).
Irradiation can directly cause DNA damage, such as single-strand breaks (SSBs), double-strand breaks (DSBs), DNA cross-links, and DNA-protein cross-links, resulting in therapeutic effects on tumor cells, such as apoptosis, necrosis, senescence, and mitotic abnormalities (53, 54). Irradiation can indirectly induce damage to DNA molecular chain in cancer cells by ionizing water molecules to generate H+ and OH- (55). This indirect effect requires oxygen. Therefore, some hypoxic tumors are resistant to radiation, which is one of the reasons for tumor recurrence after radiotherapy. Hypoxia in hypoxic tumors causes less DNA damage than in well-oxygenated tumors at the same dose of radiation. In addition, hypoxia leads to activation of the hypoxia inducible factor (HIF) signaling pathway. Activation of HIF1 can affect the expression of hundreds of genes, including vascular endothelial growth factor (VEGF) and angiopoietin-1 (ANGPT1), which promote tumor survival (56). It also drives the expression of key enzymes in glycolysis, resulting in the accumulation of lactic acid, pyruvate, and the antioxidants glutathione and NADPH to limit DNA damage (57). Therefore, radiation alone is not enough to kill all cancer cells, and it is necessary to study combination therapy.
Effects of radiation on the immune system
Traditionally, it is believed that radiotherapy leads to the death of tumor cells through irreversible damage to DNA. Many studies have found that the local killing effects of radiotherapy can be enhanced or reduced by stimulating or inhibiting the immune response in two different ways (58–60). Radiotherapy is involved in the modulation of many immune processes, such as cancer antigens release and presentation, T lymphocytes priming and activation, T cells recruitment and accumulation into tumor, T lymphocytes recognition and killing of tumor cells (61). The regimens of radiotherapy and the biological characteristics of the tumor also affect changes in immune responses.
In situ tumor vaccine induced by radiation
Radiation results in the release of DNA DSBs from tumor cells into the cytoplasm (62). Cytosolic DNA is sensed by the cyclic GMP-AMP synthase stimulator (cGAS–STING) pathway of interferon genes. cGAS is a pattern recognition receptor that triggers the production of interferon I (IFN-I) through the downstream linker stimulator of interferon genes (STING) (63–65). IFN-I can stimulate dendritic cells (DCs) and T cell activation. This is critical for converting tumors into in situ vaccines (66). There is clinical evidence that IFN-I signaling is activated in spontaneously retreating tumors (67) and in metastases highly infiltrated by T cells (68, 69).
Radiation can induce immunogenic cell death (ICD), which can induce (local and/or systemic) release of tumor-associated antigens (TAAs), especially tumor neoantigens (TNAs) (70, 71). ICD is defined as a type of regulated cell death characterized by the release of damage-associated molecular patterns (DAMPs) after cells lose membrane integrity. DAMPs include calreticulin (CRT), the chromatin stabilization protein high-mobility group box 1 (HMGB1), adenosine triphosphate (ATP), and chaperons of the family of heat shock protein (e.g. HSP70) (72, 73). ICD leads to an adaptive immune response by favoring DC cross-presentation of tumor antigens to T cells. This can enhance anti-tumor immune responses and improve tumor control (72).
DAMPs and cytokines play important roles in radiation-induced ICD. First, calreticulin (CRT) is translocated from the endoplasmic reticulum to the cell surface and can act as an “eat-me” signal to antigen-presenting cells (APCs) (especially DCs and macrophages), via binding CD91 (a 2-macroglobulin receptor) (74, 75). This induces the subsequent release of cytokines, such as interleukin-6 (IL-6) and tumor necrosis factor alpha (TNF-α) (76). The CRT-CD91 interaction also mediates the recruitment of APCs to tumors, followed by DC phagocytosis of tumor cells and efficient presentation of tumor antigens to T cells. This ultimately leads to the activation of anti-tumor immune responses (77). Radiation can further enhance the endocytic activity of APCs by interfering with the CD47-signal regulatory protein α (SIRPα) phagocytic checkpoint pathway (78–81). CD47 is a marker of self-”don’t eat me signal”, and its loss on senescent or damaged cells leads to homeostatic phagocytosis (82). Importantly, CD47 is overexpressed in many tumors, and CD47 blockade has been identified as an attractive immunotherapeutic target (83, 84). Radiation induced loss of CD47 has been reported to enhance immune-mediated tumor clearance (78). Second, high-mobility group box 1 (HMGB1) is released from dying, necrotic, damaged tumor cells into the immune milieu and exerts robust immunomodulatory effects by binding to Toll-like receptor (TLR)-4 and TLR-9 (85, 86). HMGB1 can promote DCs maturation and migration to lymph nodes for antigen cross-presentation to naive T cells (87). Third, the release of ATP, which binds to the purinergic receptor P2X7, acts as a “find me” signal for monocytes and DCs, leading to the activation of NLRP3/ASC/caspase-1 inflammasome, and ultimately induce the production of IL-18 and IL-1β (88). IL-1β promotes the activation of IFN-γ-producing tumor antigen-specific CD8+ T cells (89). Fourth, HSP70 can be translocated from the cytoplasm to the extracellular matrix under conditions of radiation-induced cellular stress (90). HSP70 can activate monocytes, macrophages, and DCs by binding to CD14, CD40, CD91, Lox1 and Toll-like receptors (TLR2 and TLR4) (91). These results showed that the release of danger signals is critical for activating of antigen-presenting cells and for enhancing the immune response to tumor cells.
The cumulative effects of these molecular signals promote DCs phagocytosis of tumor cells, thereby facilitating DCs processing of tumor-derived antigens and subsequent DC-mediated cross-presentation to CD8+ cytotoxic T lymphocytes to release or induce type I interferons. Overall, radiation can induce ICD, an important pathway for activating antitumor immunity, which can transform tumors into an “in situ vaccine”.
Abscopal effect induced by radiation
In 1953, the abscopal effect was first described as the regression of unirradiated tumors in a patient receiving radiation therapy (92). Over past decades, the abscopal effect is of great interest among radiation oncologists, but it remains a rare and poorly understood phenomenon in the clinic. In the era of cancer immunotherapy, many studies have found that radiotherapy combined with immunotherapy can enhance the abscopal effect (93–95). The key mechanism of this abscopal effect is radiation-induced in situ vaccination through liberating TAAs (7, 96). These neoantigens are then taken up by APCs, which are involved in the cross-priming of naive CD8+ T cells. Activated tumor-specific CD8+ cytotoxic T cells can move to the primary tumor and the metastatic lesions, activate systemic immunogenicity, induce abscopal effects, and control the growth of irradiated and non-irradiated tumors (60, 97).
Reprogramming the tumor microenvironment through radiation
The tumor microenvironment (TME) is the internal environment on which tumor survival and development depends, and is associated with tumor growth, progression, and metastasis (98, 99). The dynamic changes in the TME lead to tumor cell variant selection. This results in the complexity of cancer heterogeneity and influences responses to different therapeutic strategies (100–102). TME can be segregated into four immune phenotypes based on tumor mutational burden and the presence of an inflammatory gene signature enriched for IFN-γ response genes (103). Chen et al. (61) classified TME into three types: an immune-inflamed, an immune-deserted and an immune-excluded. The TME of inflamed type, a “hot” phenotype with highly infiltration of CD4+ and CD8+ T cells, is accompanied by myeloid cells and monocytic cells. In addition, the immune cells are located in proximity to the tumor cells. Excellent responses to anti-PD-L1/PD-1 agents are most often in patients with inflamed tumors (104–106). On the contrary, the TME of deserted type refers to a “cold” phenotype lacking T lymphocytes infiltration in either the parenchyma or the stroma of the tumor. These deserted tumors rarely respond to therapeutic PD-L1/PD-1 antibodies (104). The TME of the immune-excluded type is an intermediate state characterized by the presence of abundant immune cells. However, the immune cells do not penetrate the tumor parenchyma, but instead remain in the stroma surrounding tumor cell nests (107, 108). Clinical responses are uncommon after anti-PD-L1/PD-1 treatment of these immune-excluded tumors. There is evidence of stroma-associated T cell activation and proliferation, but no infiltration (109). It is not clear how radiotherapy induces an immune-activating TME and radiotherapy leads to an immunosuppressive TME.
Immune-enhancing effects of radiation in TME
More and more evidences indicated that radiotherapy can enhance innate and adaptive immune responses to tumors, thereby enhancing tumor responsiveness to radiation (110–113). Radiation therapy can induce the in situ tumor vaccine, thereby promoting the activation and maturation of DCs. DCs take up TAAs from damaged tumor cells and move to draining lymph nodes, and then present TAAs to T cells. Activated T cells can move to tumors to kill tumor cells. In addition, radiotherapy can upregulate the NK pathway to mediate tumor cells killing.
The in situ vaccination effect of radiation contributes to the uptake, processing and presentation of TAAs by DCs (such as CD11c+CD11b+ APCs) (114, 115). DCs (specialized APCs) can cross-presenting extracellular antigens, especially cell-associated antigens, to CD8+ T cells (116, 117). Many studies have shown that radiation can increase the levels of tumor-associated DCs, enhance the mobilization of these cells into draining lymph nodes, augment DCs maturation, and promote the ability of DCs (59, 60, 118). CD40 agonists are known to enhance DC function by increasing the surface expression of major histocompatibility complex (MHC) molecules and the production of proinflammatory cytokines (119). The cross-priming process requires the cognate T-cell receptors (TCR) to recognize the peptide major histocompatibility complex (MHC), which requires the costimulatory molecules CD80/86-CD28/cytotoxic T lymphocyte-associated protein 4 (CTLA4) and CD40L-CD40. Radiation can upregulate MHC-I molecules on tumor cells, thereby enhancing TAA presentation (120). This enhances tumor cells recognized by cytotoxic T cells specific to tumor antigen, and lysis of tumor cells by cytotoxic T cells. Radiation induces an increase in MHC I antigen presentation through three different mechanisms: (1) a proteasome-dependent increase in cytosolic peptide levels; (2) activation of the mTOR pathway leads to increased translation of proteins; (3) an increased generation of radiation-specific peptides (120). In addition to these cell intrinsic mechanisms of MHC-I induction, radiation-induced IFN-γ induces MHC-I upregulation (121). Therefore, radiation can increase MHC-I levels in some tumors with low endogenous MHC-I, thereby increasing immune-mediated attack. Furthermore, radiation upregulates the NK pathway by activating natural killer group 2D (NKG2D) ligands, and increasing NK cell cytotoxicity, tumor infiltration, and the production of many cytokines (112, 122). In addition, radiation can upregulate Fas expression by tumor cells, resulting in increased cytotoxic T cell lysis through a Fas/FasL-dependent mechanism (123). Radiation can induce the expression of cytokines and chemokines, such as CXC-motif chemokine (CXCL) 9, CXCL10, CXCL11 and CXCL16, thereby promoting the recruitment of effector CD8 and T-helper 1 CD4 T cells (124, 125). Radiation induces increased expression of vascular cell adhesion molecule 1 (VCAM-1) and intercellular adhesion molecule 1 (ICAM1) in tumor vessels, thereby promoting tumor infiltration by T lymphocytes (7, 126). Many studies have indicated that the presence of tumor-infiltrating lymphocytes, especially effector T cells, before therapy is associated with better survival in cancer patients (127, 128). Anitei et al. found that the densities of CD3+ T cells and cytotoxic CD8+ T lymphocytes were significantly correlated with disease-free survival and overall survival in patients with rectal cancer treated with chemoradiotherapy (129). Therefore, radiation induces the release of chemokines that subsequently enrich the T−cell infiltrate, and enhance priming of infiltrating T cells, thereby providing a positive immunological outcome.
It is clearly that radiation can act on multiple tumor compartments to stimulate the tumor immune system. The antitumor immune-enhancing effects of radiotherapy were shown in Figure 1.
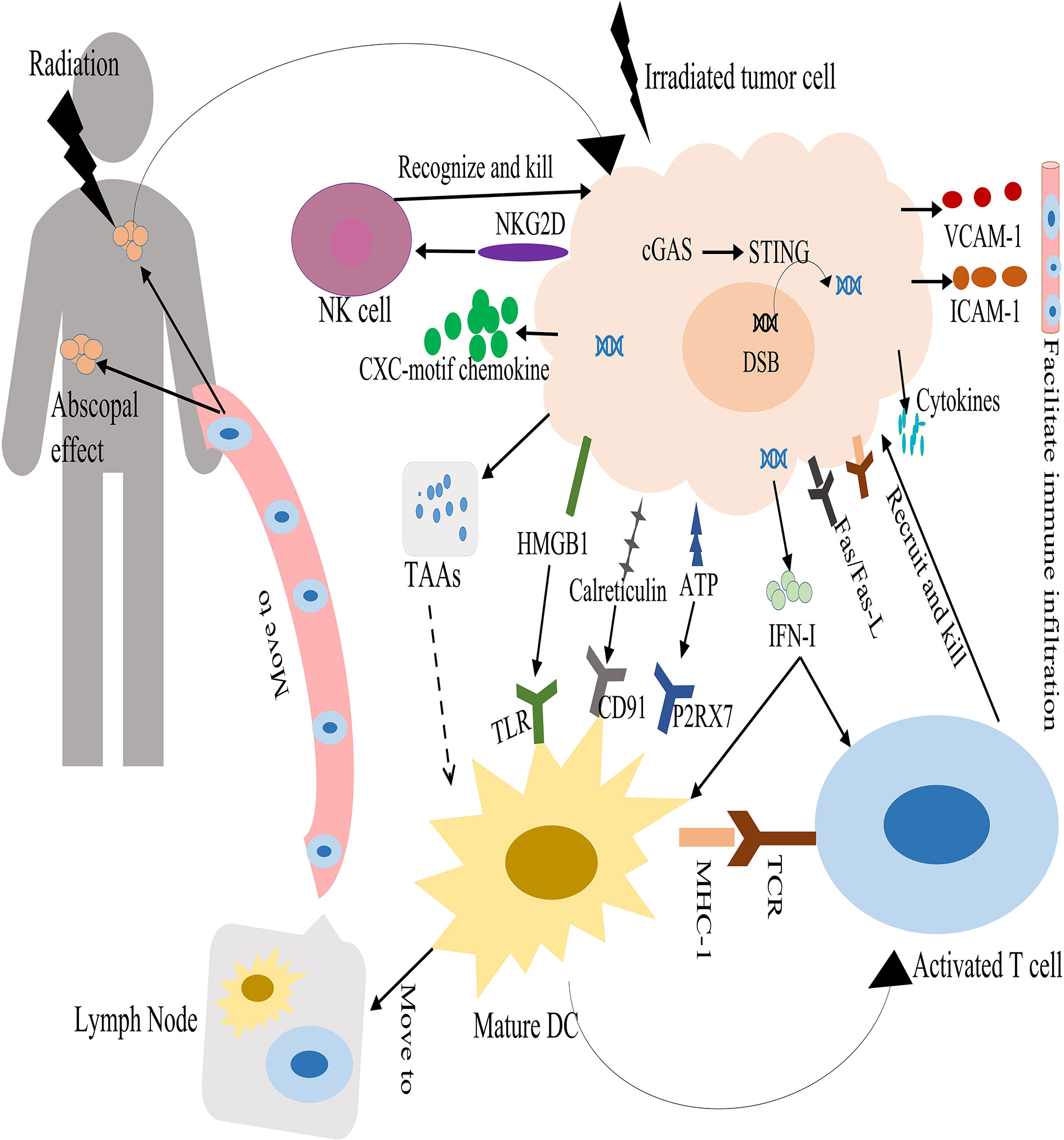
Figure 1 Antitumor immune enhancement of radiotherapy. Radiation therapy causes DNA DSBs in tumor cells and is sensed by the cGAS–STING pathway, resulting in the production of interferon I (IFN-I). In turn, IFN-I can stimulate dendritic cells (DCs) and T cell activation. Radiation therapy can induce immunogenic cell death. This releases danger-associated molecular patterns that promotes the activation and maturation of dendritic cells. DCs take up tumor-associated antigens (TAAs) from damaged tumor cells and travel to draining lymph nodes, and then present the TAAs on major histocompatibility complex class I (MHCI) to T cells through the T-cell receptor (TCR). Activated T cells move to the irradiated tumor and non-irradiated tumors through the blood circulation. Radiation can upregulate Fas and MHC-I molecules expression on tumors, and increase the release of cytokines and chemokines by tumor cells. This promotes the recruitment of activated T cells to kill tumor cells. In addition, radiation can upregulate the NK pathway to mediate tumor cells killing.
Immunosuppressive effects of radiation in the TME
In addition to modulating the TME to generate antitumor immune responses, radiation can lead to immunosuppression of the TME and induce the expression of molecules that prevent DCs from cross-presenting tumor antigens and/or T cells to kill tumor cells. It is necessary to study this topic, because the suppression of the immune microenvironment leads to worse prognosis, and it may also be a legitimate therapeutic target.
Many studies have shown that radiation can lead to the recruitment of regulatory T cells (Tregs), myeloid derived suppressor cells (MDSCs) and tumor associated macrophages (TAMs) in the tumor microenvironment (123, 130, 131). Tregs are a subset of CD4+ T cells characterized by the expression of the transcription factor fork head box P3 (FOXP3). Tregs produce the cytokines transforming growth factor beta (TGF-β) and IL−10. This suppresses effector-T−cell activation and stimulates the suppressive functions of MDSCs (132). These results indicate that Tregs in tumors develop enhanced immunosuppressive properties after radiotherapy. Many studies indicate that the presence of highly suppressed Tregs in the circulation may represent a highly immunosuppressive environment induced by chemoradiotherapy, at least temporarily, in patients with glioblastoma and head and neck or cervical cancer (133–135). Therefore, targeting Tregs and/or their immunosuppressive effector molecules may be the key to reversing immunosuppression (136–138). After radiation therapy, the increased MDSCs can suppress the activation of both CD4+ and CD8+ T-cell responses in the TME via secretion of arginase-1 (ARG1) and nitric oxide synthase 2 (NOS2) (139, 140). In addition, MDSCs promote blood vessel formation and tumor regrowth (141, 142). Many studies in a variety of tumor models have shown that radiotherapy induces the recruitment of macrophages into tumor sites (123, 143). Radiation-induced recruitment of TAMs was dependent on increased expression of the chemokine CSF-1 (144). Although M1 macrophages can promote inflammation and antitumor immune responses, the M2 phenotype can promote tumor growth, angiogenesis, and metastasis after radiation (145, 146). Irradiated tumor cells release oxygen and nitrogen radicals that promote the polarization of macrophages from an inflammatory M1 phenotype into a tumor-supporting M2 phenotype. These M2 macrophages secrete the anti-inflammatory cytokines IL-10 and TGF-β, as well as the enzyme arginase-1, which lead to T cell suppression (147, 148). TGF-β can promote extracellular matrix production and angiogenesis, resulting in tumor cell proliferation, adhesion and metastasis (149, 150). TGF-β can impede anti-tumor immunity post-radiation by suppressing the effector functions of T-cells and natural killer cells, inhibiting DC maturation, promoting M2 macrophage polarity and the conversion of CD4 + T-cells into immunosuppressive Tregs (151). Radiation can stimulate the upregulation of immune checkpoint inhibitory molecules, such as programmed cell death ligand 1 (PD-L1) on tumor cells and PD-1 or CTLA-4 on cytotoxic T cells (CTLs). This can directly inhibit cytotoxic immune cell effector functions (152, 153). Therefore, when radiotherapy is combined with immune checkpoint inhibitors (such as anti-PD-1 antibody, anti-PD-L1 antibody and anti-CTLA4 antibody), T cell activity directed against tumor cells can be increased.
In summary, radiation can promote the recruitment and activation of DCs and cytotoxic T cells through a variety of mechanisms, but this may be counteracted by the migration of suppressive immune cells. This presents an opportunity to combine radiation with immunomodulatory agents to improve tumor control. The immunosuppressive effects of radiotherapy were shown in Figure 2.
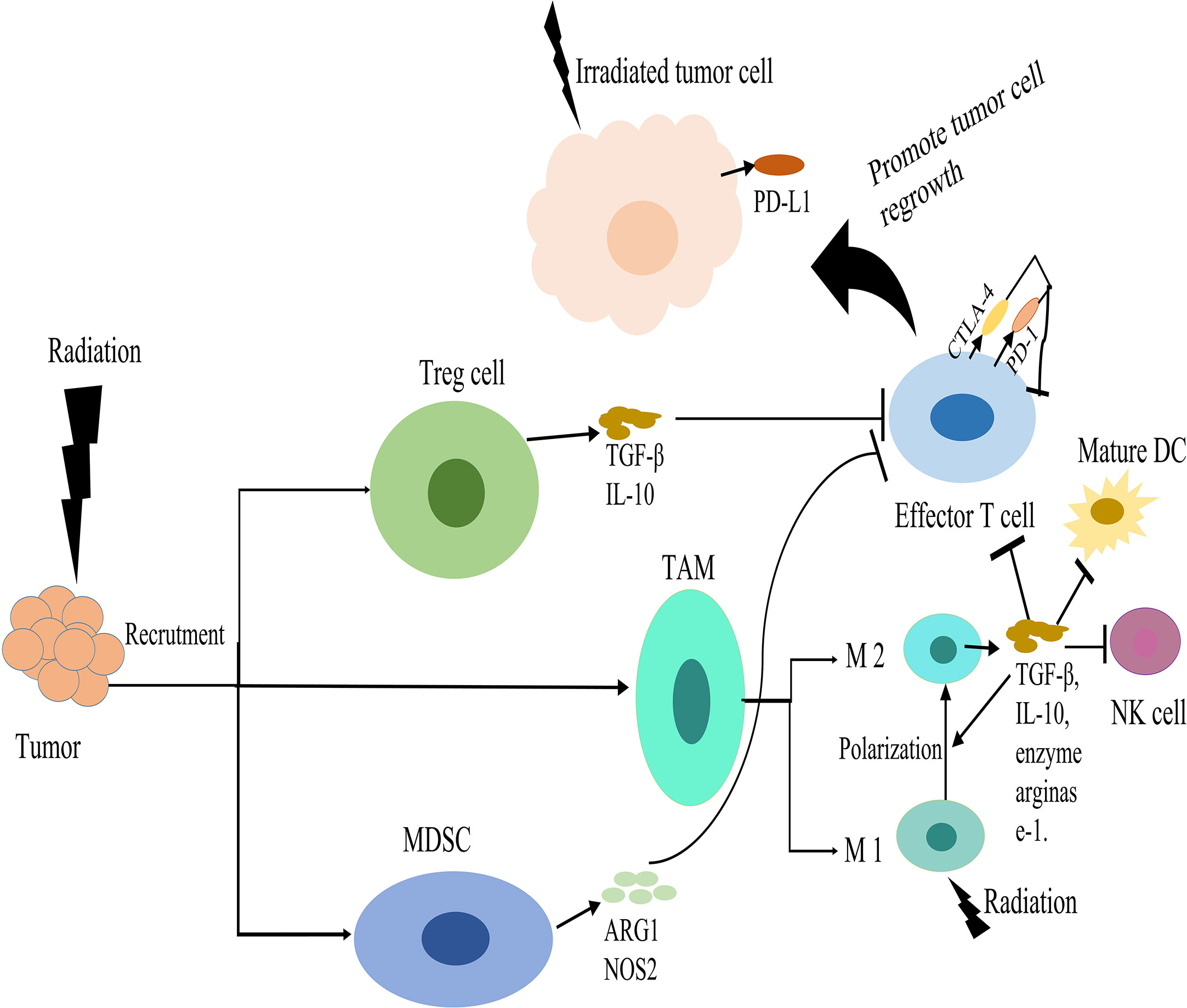
Figure 2 Immunosuppressive effects of radiotherapy. Radiation induces the recruitment of regulatory T cells (Tregs), myeloid derived suppressor cells (MDSCs) and tumor associated macrophages (TAMs) in the tumor microenvironment (TME). Tregs produce transforming growth factor beta (TGFβ) and IL-10, which suppress effector-T-cell activation. MDSCs can suppress the activation of T-cell responses via secretion of arginase-1 (ARG1) and nitric oxide synthase 2 (NOS2). Radiation can promote the polarization of macrophages from an inflammatory M1 phenotype into a tumor-supporting M2 phenotype. These M2 macrophages secrete IL-10 and TGFβ and the enzyme arginase-1, which suppress T cell. TGFβ can suppress the effector functions of T-cells and natural killer (NK) cells, inhibiting DC maturation, and promoting M2 macrophage polarity. Radiation stimulates upregulation of immune checkpoint inhibitory molecules, such as programmed cell death ligand 1 (PD-L1) on tumor cells and PD-1 or CTLA-4 on cytotoxic T cells, which down regulate T cell activation. These effects suppress the antitumor immunity and promote tumor cell regrowth.
The best combination of radiation therapy and immunotherapy
Radiation is increasingly used to control tumors locally, especially SBRT, with high rates of local control and significant benefits in terms of overall survival in many randomized trials (20, 154, 155). However, local tumor recurrence and distant tumor metastasis frequently occur when radiation therapy is used alone (156). Therefore, it is necessary to combine radiation therapy with other treatment options.
Immunotherapy has attracted great interest, and has become an established pillar of cancer therapeutics (99, 157). Unfortunately, most immunotherapeutic strategies are not effective in inducing tumor regression when used alone, and a large number of patients do not respond or become refractory to immunotherapy (106, 158, 159). The overall response rate to anti-CTLA-4 antibody is around 15%, while the response rate to PD-1/PD-L1 antibodies was < 25% (160). There are serval reasons to prevent immunotherapy from reaching its full potential. First, the priming of tumor antigen-reactive T cells is insufficient. Second, the infiltration of antitumor effectors into the tumor was weak. Third, the tumor microenvironment is highly immunosuppressive. Fourth, cancer cells effectively evade recognition by immune effectors with impaired tumor-associated antigen presentation (101, 161, 162). It is a great clinical challenge to overcome immunotherapy resistance.
Many studies showed that radiotherapy and immunotherapy are complementary. Irradiated tumors exhibit distinct patterns of immunogenicity, thereby improving response to immunotherapy (93). In addition, tumors with immunotherapy are more sensitive to radiotherapy. This can promote the localized treatment of tumors. Many studies found that radiation with ICIs can successfully treat metastatic cancers. This not only induced a local response, but also significantly regressed distant lesions outside the irradiation field (163–167). Theelen et al. pointed out that the addition of radiotherapy to pembrolizumab immunotherapy significantly improved abscopal responses and survival in patients with metastatic non-small-cell lung cancer (NSCLC), compared with pembrolizumab alone (29). This indicates that radiotherapy can convert non-responders to ICIs into responders.
Although radiotherapy combined with immunotherapy can improve the immune response, not every combination of radiation and immunization has been validated in clinical trials (32, 168). To further improve the anti-tumor ability, we need to select the appropriate patient population, explore the optimal radiotherapy regimens (dose, fractionation and volume), immunotherapy regimens (such as CTLA-4 inhibitors and PD-1/PD-L1 inhibitors), the sequence of radiotherapy and immunotherapy, and reduce the immunosuppressive effects and toxicity of radiotherapy.
The appropriate radiotherapy regimens in combination with immunotherapy
Radiotherapy is a double-edged sword that is associated with immune activation and immune suppression. Therefore, it is necessary to study the optimal dose and fraction of radiotherapy to achieve optimal anti-tumor effects in combination with immunotherapy.
High-dose radiotherapy promotes tumor immunogenicity
Many studies have shown that HDRT (such as SBRT) in combination with ICIs is more likely to cause tumor cell necrosis, enhance anti-tumor immunity, and lead to significant tumor control (94, 169). HDRT has showed a more potent immunogenic effect against cancer cells than conventional radiotherapy (usually 1.8–2 Gy per day) (23, 25). The conventional radiotherapy usually lasts for several weeks. Therefore, lymphocytes can be rapidly cleared from the irradiated field, reducing tumor antigen-specific T cell populations through sustained site-specific cytotoxicity. HDRT takes advantages over traditional radiation therapy when combined with immunotherapy.
Exposed tumor to a radiation dose ranging from 5 and 12 Gy per fraction, the number of infiltrated CD8+ cytotoxic T cells and NK cells were increased, while the number of Tregs was decreased. This is associated with the release of more anti-cancer cytokines, such as IFN-γ and TNF-α, and less immune suppressor cytokines, such as TGF-β and IL-10 (93, 170). Morisada et al. used hypo-fractioned radiation (8Gy*2f) or low-dose daily fractionated radiation (2Gy*10f) combined with anti-PD-1 antibody to treat mice bearing established syngeneic MOC1 oral carcinomas or MC38-CEA colon adenocarcinomas. They found that high-dose and low-dose fractionated radiation alone showed similar primary tumor control. However, anti-PD-1 antibody plus 8Gy*2f radiation rather than 2Gy*10f radiation, statistically significant enhanced CD8+ cell dependent primary and abscopal tumors control by inducing expression of IFN and IFN-responsive genes on tumor cells (171). Lan et al. compared ablative hypo-fractionated radiotherapy (AHFRT, 23Gy/2f/9d) versus conventional fractionated radiotherapy (36Gy/9f/9d) in mice bearing tumors from Lewis lung carcinoma or melanoma B16F10 cells, under the same conditions with biological equivalent dose (BED). They showed that AHFRT combined with anti-PD-L1 antibody presented a superior efficacy in controlling tumor growth and augmenting systemic anti-cancer immunity. The mechanism is that AHFRT suppressed the recruitment of MDSCs into tumors by regulating the VEGF/VEGFR axis, reduced MDSC-associated PD-L1 expression and increased the cytotoxicity of CD8+ T cells (25).
Many studies have shown that the abscopal effect was mainly observed in the combination of hypo-fractionated radiation regimens with ICIs. Dewan et al. used TSA and MCA38 cells to construct mouse tumor models. They found that fractionated radiation (8 Gy*3f or 6 Gy*5f) combined with anti-CTLA-4 antibody rather than single-dose radiation (20 Gy*1f) can induce an abscopal effect. In addition, 8 Gy*3f was more effective than 6 Gy*5f in eliciting systemic anti-tumor immunity combined with anti-CTLA-4 antibody (172). They further found that 20 Gy and 30 Gy single dose can attenuate cellular immunogenicity by inducing the DNA exonuclease Trex1 in various cancer cells, thereby degrading cytoplasmic DNA in irradiated cells (24). Cytosolic DNA stimulates secretion of IFN-β by cancer cells following activation of the DNA sensor cGAS and its downstream effector STING, which mediates optimal in situ vaccination (173). In fact, it was observed that the higher the dose per fraction, the more Trex1 was induced, resulting in significant DNA degradation. Therefore, the fractionated dose above the threshold (varies between 12 and 18 Gy in different cancer cells) for inducing Trex1 can result in downstream abrogation of IFN-β production, reducing DC recruitment and activation. Finally, it fails to induce systemic antitumor immune response (24). These results provide references for better selection of the radiotherapy regimens. However, these results also need to be validated clinically.
However, like conventional radiotherapy, HDRT can suppress tumor-reactive immunity by increasing the infiltration of Tregs and MDSCs, inducing an M2-like phenotype, and releasing TGF-β and IL-10 (143, 174). Lin et al. studied the effects of HDRT (8Gy/f) with and without anti-Gr-1 using syngeneic murine allograft prostate cancer models. They demonstrated that HDRT induced an early rise of MDSCs, followed by an increase of functionally active CD8 tumor-infiltrating lymphocytes. However, systemic depletion of MDSCs by anti-Gr-1 did not augment the antitumor immunity of HDRT because of the compensatory expansion of Treg-mediated immune suppression. This indicates that it is necessary to block MDSCs and Tregs for enhancing radiotherapy-induced antitumor immunity (33). Monjazeb et al. found that although HDRT induces an increase in CD8+ T cells and CD8+/PD1+/Ki67+ T-cells in the radiation field, HDRT may lead to a decrease in the ratio of M1/M2 macrophages in the tumor microenvironment (175). Furthermore, HDRT can inhibit the anti-tumor immune response by inducing tumor vascular damage. This can limit the infiltration of cytotoxic T lymphocytes into the tumor, and increase the hypoxic area (176, 177). Therefore, it is necessary to study the suppression of HDRT on the immune system, because it leads to poor prognosis, and it may be a reasonable therapeutic target.
In clinical practice, the combination of HDRT and immunotherapy is sometimes not superior to immunotherapy alone. Theelen et al. conducted a multicenter, randomized phase 2 study in advanced NSCLC patients who were treated with pembrolizumab either alone or after SBRT (8Gy*3f). They found that the overall response rate at 12 weeks was 18% in the pembrolizumab alone arm vs 36% in the pembrolizumab plus SBRT arm without statistical difference. In addition, no improvement in PFS or OS was achieved after the addition of SBRT (168). McBride et al. conducted another randomized clinical trial of nivolumab versus nivolumab plus SBRT (9 Gy*3f) in patients with metastatic or recurrent head and neck squamous cell carcinoma. The addition of SBRT to nivolumab did not statistically improve the objective response rate, OS or PFS, and there was no evidence of an abscopal effect in unselected patients (32). Therefore, ICIs and SBRT have synergistic local effects, but rare abscopal effects.
In conclusion, HDRT combined with immunotherapy does not always induce immune-enhancing antitumor effects and is only effective in a small subset of tumor patients. Tumor progression can still occur even if the complete remission is achieved. It is necessary to explore the best comprehensive treatment strategy.
Low-dose radiotherapy reverses tumor-suppressing immune system
Although HDRT in combination with ICIs shows promising efficacy for clinical application, the treatment outcome still needs to be further optimized. A recent theory proposed that LDRT can modulate the TME, perhaps revolutionizing tumor treatment efficacy. LDRT usually refers to doses below a threshold, that is, the amount of doses less than that can physically damage DNA or kill cancer cells directly (178). The most common LDRT doses are 0.5-2 Gy/fraction, with total doses up to 1-10 Gy (179, 180). According to previous reports, LDRT modulated the immune suppressive stroma by downregulating TGF-β, repolarizing macrophages to favor the M1 over the M2 phenotype, and significantly enhancing the infiltration of effector CD4 T cells and NK cells. LDRT improved the efficacy of anti-PD1 and anti-CTLA4 agents, thereby promoting the overall systemic antitumor response (41). We will describe the ways that LDRT modulates the immune system in detail.
First, LDRT promotes the differentiation of macrophages to an M1-like phenotype. M2 macrophages can suppress antitumor immunity, and promote a radioresistant phenotype by secreting immunosuppressive mediators, such as IL-10 and TGF-β (181). Therefore, transforming the type of macrophages is critical to improve the immune enhancing effect. Felix Klug et al. (179) demonstrated that LDRT (0.5-2 Gy) can effectively transform M2 macrophages to iNOS+ M1 phenotypes, resulting in strong CD4+ and CD8+ T cells infiltration into human pancreatic carcinomas. After application of 0.5 Gy, the irradiated tumors contained the highest number of T cells, accompanied by an increase in CD4+ FoxP3+ T cells. In addition, LDRT can induce vascular normalization through crosstalk between macrophages and T cells. LDRT promoted T cell-mediated tumor eradication and prolonged survival (179). Prakash et al. irradiated advanced tumor-bearing Rip1-Tag5 mice with LDRT (2Gy*2f). They found profound changes in the inflammatory tumor microenvironment, characterized by induction of M1-related effecter cytokines as well as reduced cytokines of tumor-promoting and M2-related effecter cytokines (182). Furthermore, LDRT can program macrophages differentiation to an M1-like phenotype by ameliorating the hypoxia problem of tumors. Tumor hypoxia is known to be performed by angiogenesis-promoting HIF-1. This promotes angiogenesis, thereby interfering with tumor infiltration of CD8+ T cells and retuning of M1 phenotypic macrophages across the inert endothelium. Finally, it results in immunosuppressive effects (183–185). Nadella et al. demonstrated that LDRT (2 Gy) can downregulate HIF-1 in irradiated tumors, thereby supporting the differentiation of naive macrophages toward the M1 phenotype (186). Therefore, solving the hypoxia problem of bulk tumors can enhance the immune efficacy. LDRT has also been clinically observed to promote the differentiation of M1-type macrophages (175). Monjazeb et al. conducted a multicenter phase 2 study of 20 patients with histologically confirmed metastatic microsatellite-stable colorectal adenocarcinoma who had received at least one line of chemotherapy. These patients were randomly assigned to repeated LDRT or HDRT with PD-L1/CTLA-4 inhibition. They found that LDRT has the potential to increase the ratio of M1/M2 macrophages (175).
Second, LDRT can promote anti-tumor cytotoxicity of NK cells. LDRT can augment the direct expansion and cytotoxicity of NK cells through the P38-MAPK pathway (187). In addition, Sonn et al. found that when purified NK cells were irradiated with 0.2 Gy, the toxicity of NK cells was enhanced, while cell proliferation and apoptosis were unaffected (188). Cheda et al. (189) compared BALB/c mice that received or did not receive LDRT (single dose of 0.1 or 0.2 Gy), which were then injected with sarcoma cells. They found that the number of pulmonary tumor colonies was significantly reduced, and the cytolytic function of NK cells was significantly stimulated in the irradiated mice compared with the control group. In addition, NK-inhibitory anti-asialo GM1 antibody can totally abolish the tumor suppressive effect of LDRT. These results indicate that LDRT suppresses the development of experimental tumor metastases by stimulating the cytolytic function of NK cells.
Third, LDRT enhances T-cell infiltration. Herrera et al. (190) reported that LDRT of murine tumors promoted T-cell infiltration and responded to combinatorial immunotherapy in an IFN dependent manner. The mechanism is that LDRT induces CD4+ cells with characteristics of exhausted effector cytotoxic cells. One subset expressed NKG2D and exhibited proliferative capacity, as well as a unique subset of activated DCs expressed the NKG2D ligand RAE1. Zhou et al. established an in vivo lung cancer model. They found that LDRT activated T cells and NK cells, and promoted splenocyte cytotoxicity and T cell infiltration in the tumor tissues (191). Hashimoto et al. found that low-dose total body irradiation (0.2 Gy) increased the proportion of CD8+ cells in splenocytes, and even tumor-infiltrating lymphocytes were predominantly CD8+. Low-dose total body irradiation (0.2 Gy) inhibited lung and lymph node metastasis in tumor-bearing rats (192). In addition, low-dose total body irradiation of 2 Gy represents a powerful tool to foster CD4+ T cell-based cancer immunotherapies by favoring T helper 1 cells driven antitumoral immunity (193).
Fourth, LDRT can affect the function and activity of regulatory T cells (Tregs), thereby enhancing antitumor immunity. Tregs belong to a group of T lymphocytes that possess a negative immune regulatory function. The increased numbers of these cells in liver, breast, and ovarian cancer are closely related to the immune escape, occurrence, and development of tumor cells. Wang et al. found that LDRT (total 0.45 Gy) of the spleen can shrink tumors and increase the survival rate of rats with liver cancer. The mechanism by which LDRT enhances the immune effects may be that LDRT reduces the ratio of CD4+CD25+Treg/CD4+ in the blood and Foxp3+, IL-10, TGF-β and cytotoxic T lymphocyte-associated antigen 4(CTLA-4) expression (194). Liu et al. found that LDRT significantly reduced the percentage and absolute numbers of CD4+CD25+Foxp3+ regulatory T cells in naive mice, whereas CD4+CD44+/CD8+CD44+ effector memory T cells were greatly increased in naive mice (195). These results indicate that LDRT is a potential approach to overcome the tumor immunosuppressive microenvironment.
Finally, LDRT enhances the efficacy of ICIs. Barsoumian et al. (41) established mouse tumor models and irradiated the tumors with different doses. They found that LDRT alone (1Gy*2f) can effectively prolong survival by controlling tumor growth. The anti-tumor efficacy was further significantly enhanced when combined with anti-PD1 and anti-CTLA-4 drugs. This may be because LDRT can significantly activate CD4 and CD8 T cells, and enhance NK cell infiltration and M1 macrophage polarization and reduce TGF-β cytokine. Nowosielska et al. (196) found that LDRT to the whole-body (0.1 or 1.0 Gy) combined with anti-CTLA-4 antibody and anti-PD-1 antibody and NVP-AUY922 significantly reduced tumorigenesis in mice, and inhibited the clonogenic potential of Lewis lung cancer cells in vitro. By using targeted radionuclide therapy to semi-selectively deliver radiation to mouse tumors, Patel et al. found that low-dose targeted radionuclide therapy enhances the response of immunologically “cold” tumors to ICIs. After the combination of targeted radionuclide therapy and ICIs, 45-66% of mice exhibited complete responses and tumor-specific T-cell memory, while only 0% with targeted radionuclide therapy or ICIs alone. The reason is that the combination therapy activates the production of proinflammatory cytokines in the TME, promotes tumor infiltration and clonal expansion of effector CD8+ T cells, and reduces spontaneous metastasis (197). Furthermore, the addition of LDRT to PD-L1/CTLA-4 blockade was feasible and safe in clinical practice (175). In a phase I clinical study, Herrera et al. found that the adding LDRT (0.5 or 1 Gy per fraction) to the combination immunotherapy group showed a therapeutic effect for an overall disease control rate of 87.5% in patients with immune desert tumors. In addition, using a single-sample gene set enrichment analysis approach, they observed that responding tumors exhibited an increase in Th1, CD8+ and TEM signatures, whereas non-responding tumors exhibited an upregulation of M2 macrophage and neutrophil signatures (190). However, in some cases, LDRT combined with immunotherapy failed to induce effective antitumor immunity. Schoenfeld et al. (198) conducted an open-label, multicenter, randomised, phase 2 trial involving 90 patients with metastatic NSCLC resistant to PD(L)-1 therapy. Patients were randomly assigned to 3 arms, durvalumab plus tremelimumab alone, or in combination with LDRT (2 Gy/4f), or in combination with HDRT (24 Gy/3f). Radiotherapy was delivered at 1 week after initial durvalumab–tremelimumab administration. They found that neither HDRT nor LDRT increased the response to combined PD-L1 plus CTLA-4 inhibition.
The rationale for using LDRT is not necessarily to ablate or kill the tumors, but to activate the immune system to eliminate these lesions in concert with other therapeutic approaches. Clinically, LDRT has the following advantages over HDRT. First, the toxicity of LDRT is low. If radiotherapy is to be delivered simultaneously to several lesions within an organ, it is difficult to meet the dose limit to the organ at risk with SBRT, whereas dose limits will be easier to meet with LDRT. Therefore, LDRT is dosimetrically more feasible than SBRT in the treatment of large tumor volumes, or even a whole organ. Second, LDRT is safer for patients who have received radiation in the past. There is a minimal concern about exceeding normal tissue dose-constraints when re-radiation is performed by LDRT. Finally, LDRT is easier to be delivered. In clinical practice, LDRT can be performed through three-dimensional technology, while HDRT requires specialized imaging, respiratory gating, and even gold fiducials implantation.
In sum, LDRT provides an emerging approach to address limitations of radioimmunity mechanisms. It is necessary to further study this important method Immunomodulatory effects of LDRT in tumor microenvironment are shown in Figure 3.
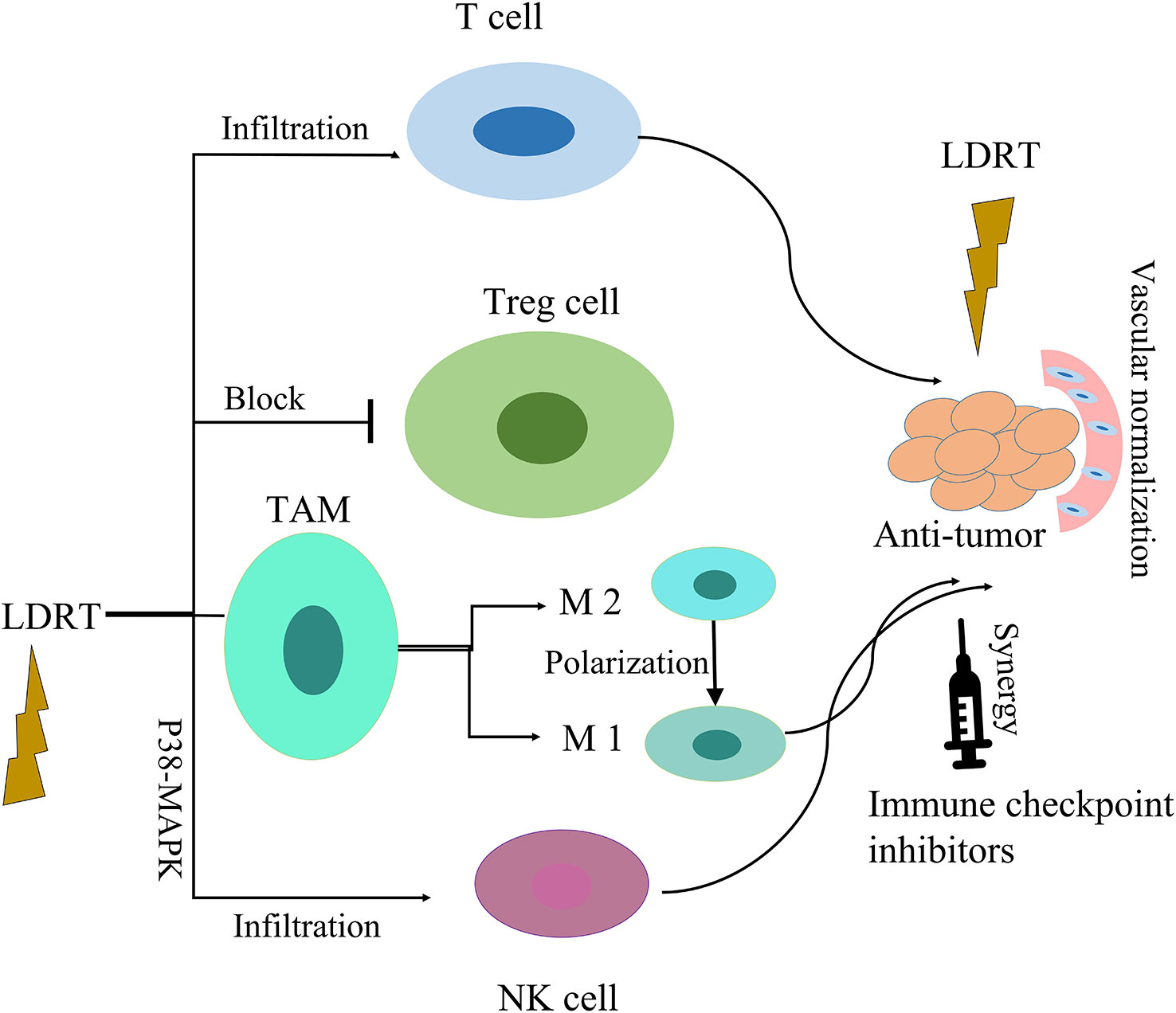
Figure 3 Immunomodulatory effects of LDRT in tumor microenvironment. LDRT modulates the tumor microenvironment by repolarizing macrophages to favor the M1 over the M2 phenotype, blocking regulatory T cells, and enhancing the infiltration of effector CD4 T cells and NK cells. Low-dose radiation can improve the efficacy of immune checkpoint inhibitors.
High-dose and low-dose radiotherapy synergistically enhance antitumor immune responses
HDRT and LDRT work differently on the immune system. We take full advantage of the advantages of HDRT and LDRT to enhance anti-tumor immune responses. HDRT can increase the release and presentation of tumor antigen, and stimulate immune cell activation. However, LDRT can modulate the TME to stimulate immune cell infiltration into the tumor stroma and the tumor bed of distant tumors. Next, we will introduce the preclinical and clinical studies of HDRT combined with LDRT, i.e., multimodal radiotherapy.
Studies showed that HDRT in combination with LDRT was superior to HDRT or LDRT alone in tumor control and activation of anti-tumor immunity (39, 199). Savage et al. (39) designed a novel radiation scheme (PAM-RT), a single high-dose radiation (22Gy) followed by post-ablation modulation with four daily low-dose fractions (0.5Gyx4f). They found that PAM-RT localized to the primary tumor in 3LL tumor-bearing mice can significantly delay tumor growth and increase survival. They treat metastatic breast cancer (4T1) mice with PAM-RT, where the primary tumor received high-dose irradiation (20 Gyx3f) and metastatic organs received low-dose irradiation (whole lung, 0.5 Gyx4f). Survival was significantly increased after whole-lung radiated by low dose compared with primary tumor ablative radiotherapy alone. The mechanism is that PAM-RT can promote remodeling of the TME in the primary tumor as well as the metastatic site by reducing Tregs, activating macrophages to an inflammatory phenotype, and promoting infiltration of CD8+ CTLs into metastatic tumors. Liu et al. (199) used a combination of hypo-fractionated radiation therapy (8Gy×3f) targeted primary tumor with low-dose total body irradiation (0.1Gy) in a syngeneic mouse model of breast or colon carcinoma. They found that low-dose total body irradiation alone did not delay the growth of primary or secondary tumors. Hypo-fractionated radiation therapy led to a significant growth delay of the irradiated primary tumors, but did not have a systemic immune related response to secondary tumors. However, the combination of low-dose total body irradiation and hypo-fractionated radiation therapy significantly delayed the growth of both the primary and secondary tumors, and translated into the best OS with systemic antitumor response characteristics. The mechanism is that the combination therapy (HDRT and LDRT) induced infiltration of CD8+ T cells, IFN-γ+ CD8+ T cells and DCs in unirradiated tumors, reduced granulocytic-myeloid-derived suppressor cells and M2 macrophages, and increased the percentage of antitumor eosinophil population. These results indicate that LDRT can serve as a potential therapeutic agent for patients with metastatic cancer. Their therapeutic potential is significantly enhanced when combined with HDRT.
Compared with the combination of ICIs with either LDRT or HDRT alone, the combination of LDRT and HDRT further enhanced the response to ICIs, resulting in an enhanced antitumor response (40–42, 197). Barsoumian et al. proposed the use of high dose and low dose radiation (RadScopal technique) with immune oncology agents (anti-TIGIT and anti-PD1 monoclonal antibodies) to against highly metastatic lung adenocarcinoma tumors in 129Sv/Ev mice. They found that the triple therapy can prolong the survival of treated mice, and halt the growth of primary and secondary tumors,and reduce the percentages of TIGIT+ exhausted T-cells and TIGIT+ regulatory T-cells (40). Yin et al. (42) compared HDRT/anti-PD1, HDRT/LDRT, or LDRT/anti-PD1 double treatments. They demonstrated that the enhancement of the abscopal response was achieved by triple therapy consisting of HDRT (8 Gy*3f) to treat the primary tumor, LDRT (2 Gy*1f) to treat the abscopal tumor, and anti-PD1 therapy. The enhanced abscopal effect was associated with increased infiltration of CD8+ effector T cells and upregulated expression of T cell-attracting chemokines. The triple treatment also improved the tumor response in patients with metastatic NSCLC and was well tolerated. In addition, HDRT combined with LDRT and double agent checkpoint blockers can effectively control metastatic tumors by increasing CD4+ effector T cells, enhancing NK cell activation, and increasing M1 macrophages in secondary tumors. Further clinical studies have shown that when the tumor burden was high, it was necessary to use HDRT to priming T cells at the primary tumor site, and LDRT to modulating the stroma of secondary (metastatic) tumors (41).
Surprisingly, the treatments combining HDRT with LDRT and immunotherapy have also achieved promising clinical results. Analyzing 26 cancer patients received LDRT (1-20 Gy in total), Menon et al. found that this was because of the scatter of HDRT or the intentional treatment at a second isocenter of LDRT. These patients underwent prospective clinical trials on the combination of radiotherapy and immunotherapy. They compared lesions that received LDRT with without radiation (< 1 Gy). 85% of lesions that received LDRT achieved PR/CR, while 18% of lesions that received no-dose (P=0.0001). This indicates that LDRT can increase systemic response rates of metastatic disease treated with HDRT and immunotherapy (43). They also conducted a phase II trial of ipilimumab with concurrent or sequential SBRT (50 Gy/4f or 60 Gy/10f) for metastatic lesions in the liver or lung. Some non-targeted lesions received LDRT (5–10 Gy) because they were anatomically close to another irradiated site. Further analysis showed that lesions that received LDRT were more likely to respond than those that did not (31% vs 5%, P=0.0091) (200). Patel et al. analyzed a phase II trial of HDRT (3–12.5 Gy/f up to 20–70 Gy total) with or without LDRT (1-10 Gy total; 0.5-2 Gy/f) for patients who had the metastatic disease that progressed on immunotherapy within 6 months. A total of 74 patients with NSCLC or melanoma were enrolled in the study. 39 patients received HDRT and 35 patients received the combination of HDRT and LDRT. There was no significant difference regarding disease control rate. However, the overall response rate for HDRT + LDRT vs. HDRT cohorts, lesions treated by LDRT was significantly improved rates of lesion-specific responses compared with nonirradiated lesions. This is because LDRT induced a remarkable increase of T- and NK cell infiltration into the irradiated lesions (180). These clinical studies indicate that HDRT and LDRT combined with immunotherapy can synergistically generate tumor-specific immune responses, thereby enhancing systemic antitumor effects.
In summary, multimodal radiotherapy, a combination of HDRT to stimulate T cell priming together with LDRT to modulate inhibitory tumor microenvironment, can enable immune cells to infiltrate into tumor bed and trigger antitumor responses. This provides a new treatment alternative for patients with advanced cancer after multiple lines of therapy, and brings immunotherapy into a new field of systemic disease control. Many clinical trials are investigating the efficacy and safety of different combinations of HDRT and LDRT in patients with advanced tumors (Table 1).
Challenges in clinical practice
Patient response to immunotherapy or immunotherapy combined with HDRT can be enhanced by delivering LDRT to certain tumor sites for the purpose of immunomodulating the TME, thereby promoting systemic propagation of anti-tumor immunity and the destruction of tumor by immune effector cells. However, it is necessary to further study clinical practices of high-dose and low-dose radiotherapy. For example, which tumor site should be treated with HDRT or LDRT? Whether HDRT and LDRT should be delivered to the same tumor site? What is the best sequence of HDRT and LDRT?
Selection of irradiation sites for high and low dose radiotherapy
There are few literatures on the targeting volume selection in studies combing immunotherapy with multimodal radiotherapy (HDRT and LDRT). Based on existing reports and clinical experiences, we developed individualized strategies based on performance status of patients, clinical symptoms, extent of tumor burden, and the immune type of the tumor microenvironment (Figure 4). This individualized treatment regimen can not only control the tumor, but also improve the patient’s quality of life.
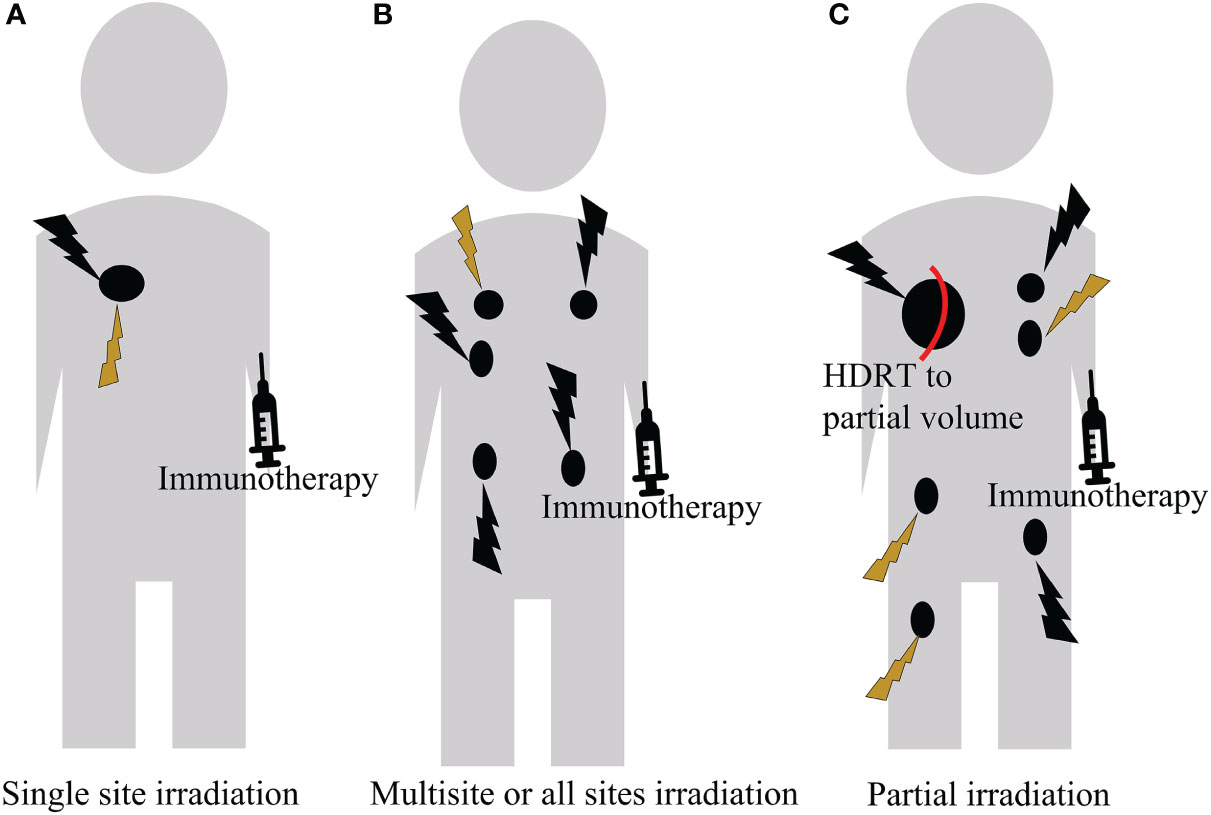
Figure 4 A personalized treatment regimen based on the patient’s performance status, clinical symptom, extent of tumor burden, and the immune type of the tumor microenvironment. (A) For a single tumor, HDRT can initiate T cell priming, followed by LDRT to modulate the tumor microenvironment. (B) For oligometastatic disease, HDRT can be delivered to all lesions. If it is infeasible and intolerable, it can be supplemented with LDRT. (C) For extensive metastatic disease, partial volume HDRT can be delivered to one or a few lesions, followed by LDRT to other lesions.
Single site irradiation
For patients with only a single lesion, HDRT can be used to ablate the tumor for achieving the radical cure. This not only shrinks the tumor. but also promotes the release of antigens. Subsequent application of LDRT to modulate the tumor microenvironment can attenuate the immunosuppressive effects of SBRT, and increase immune effector cell infiltration, thereby synergistically enhancing the response to ICIs. However, it is necessary to study it in the future. At present, only a preclinical study has reported that HDRT followed by LDRT to the primary tumor can delay tumor growth and prolong survival in mice (39).
Multisite or all sites irradiation
Tumors are heterogeneous (201, 202). This indicates that tumor associated antigens (TAAs) present in some tumor sites may be different from those in other tumor sites, or may not be equally immunogenic. Targeting a single metastatic site in patients with multiple metastases cannot unmask TAAs in another site unless those TAAs are shared. Therefore, useful antitumor immune responses are not activated systemically.
Monjazeb et al. (175) did not observe objective responses outside the irradiation field. This indicates that irradiating 1-2 lesions in combination with the immune checkpoint blockade is not sufficient to mediate systemic antitumor immunity in patients with refractory colorectal cancer. In addition, in the multicenter, randomized phase 2 study, 76 patients with recurrent metastatic NSCLC were randomized to either pembrolizumab alone or pembrolizumab to a single tumor site after SBRT (3 doses of 8 Gy). The overall response rate of the added SBRT group was twice that of the control group. However, the results did not meet the criteria of a meaningful clinical benefit endpoint (168). Luke et al. conducted a phase I study enrolling patients with metastatic solid tumors who had progressed on standard treatment. 69 patients were treated with SBRT (total of 30-50Gy/3-5f) and at least one cycle of pembrolizumab. SBRT was delivered to two to four metastases, but not all metastases were irradiated. They found that multi-site SBRT can limit the progression of existing metastases, and enhance anti-tumor immune responses. This improves outcomes in metastatic patients treated with pembrolizumab (203). Iyengar et al. (21) found that the addition of SABR to all severe disease sites significantly improved PFS without increasing toxic effects. In addition, studies have reported that multisite SBRT followed by pembrolizumab was safely tolerated (27, 204). These clinical experiences indicate that irradiation of multiple sites or all metastatic lesions can maximize systemic synergy.
Patients classified as having oligo-metastases may be candidates for HDRT delivery to all lesions for immune priming and local control. In addition, direct delivery of SBRT to all tumor sites ensures tumor sterilization. However, irradiation of all lesions by HDRT may sometimes be infeasible and intolerable in clinical practice because of dose volume constraints in normal tissue surrounding the tumors. Therefore, some tumor lesions can be supplemented with low-dose irradiation.
In sum, multiple target irradiation tends to induce stronger antitumor immunity and generate more frequent abscopal responses than a single target. Therefore, it is necessary to further study this approach in clinical settings.
Partial irradiation
Systemic therapy is the standard of care in patients with non-oligometastatic cancers. However, the addition of SBRT can not only shrink the local tumor to induce an effect of in situ vaccination, but also relieve local symptoms, such as pain, obstruction and bleeding, etc. To enhance the systemic efficacy of immunotherapy, it is necessary to use HDRT to stimulate immune priming for the bulky or “cold” tumors. The partial irradiation may be considered in this case because of toxicity.
Preclinical experiments have shown that high-dose partial irradiation can delay tumor growth through immune activation. Markovsky et al. (205) treated 50% or 100% of tumors with radiation in a 67NR Murine Orthotopic Mammary Tumor Model and the less immunogenic Lewis Lung Carcinoma mouse model. They found that partial irradiation in immunocompetent mice resulted in a tumor response similar to full irradiation. This is because of the CD8+ T cell-mediated immune stimulation mechanism. Furthermore, a significant abscopal effect was elicited after hemi-irradiation of the primary tumor with a single dose of 10Gy in the 67NR model. Yasmin-Karim et al. (206) found irradiating a field smaller than the entire tumor volume showed the same or better distal effect than irradiating the entire tumor volume field, and significantly reducing healthy tissue damage. This is due to higher infiltration of cytotoxic CD8+ T lymphocytes in treated and untreated tumors.
The partial irradiation combined with immunotherapy is clinically feasible. Luke et al. found that partial irradiation by SBRT was performed when the metastases were greater than 65 mL. They compared patients with at least one tumor partially irradiated to those with fully irradiated tumors, and found no statistically significant tumor control rate at 3 months (27). They further found no statistically significant difference in objective response rate, PFS, and OS between patients who received full and partial SBRT at multiple sites in the presence of pembrolizumab. Furthermore, a clinical response at the irradiated site can be induced without irradiating the entire metastases (203). Lemons et al. (207) reported that partially irradiated tumors exhibited similar control as completely irradiated tumors in patients with metastatic solid tumors treated with pembrolizumab and SBRT. These indicate that partial volume SBRT is enough to activate immune priming.
A novel partial irradiation technique, spatially fractionated radiation therapy (SFRT), can also induce an antitumor immune response. SFRT can deliver a high dose to a large irradiation field that is segmented into several small units with steep dose gradients, which lead to reduce the normal tissue toxicity (208–211). In the study of Johnsrud et al. (212), whole tumor irradiation or SFRT (a single dose of 20 Gy) alone or in combination with ICI were tested in mice using a triple negative breast tumor. In the group of SFRT, they observed the abscopal immune response in contralateral tumors with obviously increased infiltration of both antigen-presenting cells and activated T cells, followed by an increase in systemic IFNγ production and ultimately a delay in tumor growth. Further studies are needed to explore the new partial irradiation technique.
In addition to immunotherapy for those patients with extensive metastases, it is beneficial to apply partial volume HDRT to one or several lesions to induce immunogenic cell death. Then, applying LDRT to other lesions for tumor microenvironment modulation can enhance abscopal effects, thereby reducing tumor burden.
The optimal sequence of high and low dose radiotherapy
HDRT and LDRT combined with immune checkpoint blockades can improve local and systematic antitumor responses in advanced tumors. However, the optimal sequence of these therapies for optimal efficacy remains unclear.
For combination therapy, LDRT can be applied before or after HDRT. Many existing studies about the sequencing issue have different results. Savage et al. (39) divided C57BL/6 mice with palpable subcutaneous 3LL tumors into five treatment groups: no treatment, 24 Gy on day 1 or 5, four fractions of 1Gy followed by 20 Gy or 20 Gy followed by four fractions of 1Gy, four of which were treated with radiation therapy to the primary tumor. They found that 1Gyx4f after ablation radiation (20 Gy) showed the best tumor control and the longest survival. However, pre-treatment with low-dose radiotherapy resulted in minimal tumor control compared with single-dose ablative radiotherapy. This is due to the rapid growth of 3LL tumors, and ablative radiation targeting the larger tumors on day 5. This can affect the efficacy of the priming radiation. Therefore, it is necessary to control rapidly growing tumors with high-dose radiotherapy first, and then modulate the immune microenvironment with low-dose radiotherapy. However, other scholars found that sequential administration of LDRT followed by HDRT achieved superior antitumor immunity than the start of HDRT before LDRT. Liu et al. (199) administered HDRT to the primary tumor at 48, 72, 96, and 120 h after low-dose total body irradiation (L-TBI) in mouse tumor models. Starting HDRT at 72 h after L-TBI can achieve the best overall survival and the maximum abscopal effect. In addition, they compared the time of L-TBI 3 days before or after HDRT, or simultaneously with HDRT. The results showed that HDRT 3 days after L-TBI exhibited the best therapeutic effect, for example, a significant inhibition of tumor growth and improved survival of the treated mice. They found that L-TBI followed by HDRT can induce an adaptive immune response and protect the immune system of the mice. Zhou et al. observed that LDRT pretreatment before HDRT was able to ameliorate the HDRT-induced immune impairment and enhance the antitumor immunity (191). Therefore, it is necessary to conduct large preclinical and clinical trials for the optimal sequencing of HDRT and LDRT.
However, the optimal timing of the addition of immunotherapy to HDRT and LDRT remains unclear. Some scholars pointed out that immunotherapy is more effective after radiotherapy than before. This is because radiotherapy can promote the release of TAAs and destroy any pre-existing immune tolerance in the tumor periphery. Wei et al. (213) demonstrated that the administration of αPD-1 antibody after local tumor irradiation could induce a potent abscopal response while the addition of αPD-1 before radiation abrogated the abscopal effect. This antitumor efficacy was associated with the expansion of polyfunctional intratumoral CD8+ T cells, reduction of intratumoral dysfunctional CD8+ T cells, and expansion of reprogrammable CD8+ T cells. Many studies showed that the concurrent combination of anti-PD-L1 antibody and radiation achieved better tumor control than the sequential schedule (214, 215). Bestvina et al. conducted a randomized phase 1 trial comparing the combination of nivolumab and ipilimumab with sequential or concurrent multisite SBRT in patients with stage IV NSCLC. They found that the median PFS were 18.6 and 13.2 months for concurrent and sequential therapy, respectively. The concurrent treatment strategy was not more toxic than the sequential one (216). However, there are some different views. A phase 1 trial compared combined pembrolizumab with SBRT administered either prior to the first pembrolizumab cycle (arm A) or prior to the third pembrolizumab cycle (arm B). Their results indicated that ORR of arm B was significantly better than that of arm A (44.4% vs 0%) (217). This is because the administration of immunotherapy prior to SBRT stimulates antigen-presenting cells and effector T cells, thereby making these cells ready to respond to the tumor antigen efflux generated by SBRT (169). Therefore, it is necessary to further study the optimized sequence of high and low dose radiotherapy combined with immunotherapy according to the biological characteristics of tumors, the selection of immunotherapy drugs, and the effects of radiotherapy on the immune system.
Conclusion
In this paper, we introduced a multimodal radiotherapy regimen (HDRT combined with LDRT) to synergistically enhance the local and systemic antitumor immunity, and improve the response to immunotherapy, thereby achieving the best anti-tumor effects. HDRT induces in situ tumor vaccine and primes cytotoxic T cells. LDRT modulates the tumor microenvironment, which in turn promotes the infiltration and lethality of immunocompetent cells. This multimodal radiotherapy regimen can be applied to primary tumor and metastatic lesions, thereby improving the local and systemic antitumor immunity. It is even possible to irradiate the whole organ with LDRT to boost immunity for widespread organ metastases, such as the lungs or liver. In clinical practice, it is possible to individually implement multimodal radiotherapy coupled with immunotherapy according to the patient’s performance status of patients, disease burden, and tumor immune microenvironment phenotypes. It is necessary to conduct a further study to solve those issues.
Author contributions
XS: Conceptualization. XJ, JW, SL, HH, WJ and GC searched the literature. XJ and WJ wrote the first draft. XS, WD and BZ wrote and edited overall. All authors contributed to the article and approved the submitted version.
Funding
The work was supported by grants from the Hospital Research Foundation of Jinling Hospital (YYQN2021084).
Conflict of interest
The authors declare that the research was conducted in the absence of any commercial or financial relationships that could be construed as a potential conflict of interest.
Publisher’s note
All claims expressed in this article are solely those of the authors and do not necessarily represent those of their affiliated organizations, or those of the publisher, the editors and the reviewers. Any product that may be evaluated in this article, or claim that may be made by its manufacturer, is not guaranteed or endorsed by the publisher.
References
1. Atun R, Jaffray DA, Barton MB, Bray F, Baumann M, Vikram B, et al. Expanding global access to radiotherapy. Lancet Oncol (2015) 16(10):1153–86. doi: 10.1016/S1470-2045(15)00222-3
2. Citrin DE. Recent developments in radiotherapy. New Engl J Med (2017) 377(11):1065–75. doi: 10.1056/NEJMra1608986
3. Delaney G, Jacob S, Featherstone C, Barton M. The role of radiotherapy in cancer treatment: Estimating optimal utilization from a review of evidence-based clinical guidelines. Cancer: Interdiscip Int J Am Cancer Soc (2005) 104(6):1129–37. doi: 10.1002/cncr.21324
4. Caudell JJ, Torres-Roca JF, Gillies RJ, Enderling H, Kim S, Rishi A, et al. The future of personalised radiotherapy for head and neck cancer. Lancet Oncol (2017) 18(5):e266–e73. doi: 10.1016/S1470-2045(17)30252-8
5. Grassberger C, Ellsworth SG, Wilks MQ, Keane FK, Loeffler JS. Assessing the interactions between radiotherapy and antitumour immunity. Nat Rev Clin Oncol (2019) 16(12):729–45. doi: 10.1038/s41571-019-0238-9
6. Carvalho H, Villar RC. Radiotherapy and immune response: The systemic effects of a local treatment. Clinics (2018) 73:e557s. doi: 10.6061/clinics/2018/e557s
7. Herrera FG, Bourhis J, Coukos G. Radiotherapy combination opportunities leveraging immunity for the next oncology practice. CA: Cancer J Clin (2017) 67(1):65–85. doi: 10.3322/caac.21358
8. Demaria S, Ng B, Devitt ML, Babb JS, Kawashima N, Liebes L, et al. Ionizing radiation inhibition of distant untreated tumors (Abscopal effect) is immune mediated. Int J Radiat Oncol Biol Phys (2004) 58(3):862–70. doi: 10.1016/j.ijrobp.2003.09.012
9. Janopaul-Naylor JR, Shen Y, Qian DC, Buchwald ZS. The abscopal effect: A review of pre-clinical and clinical advances. Int J Mol Sci (2021) 22(20):11061. doi: 10.3390/ijms222011061
10. Sharma P, Allison JP. The future of immune checkpoint therapy. Science (2015) 348(6230):56–61. doi: 10.1126/science.aaa8172
11. Brahmer JR, Tykodi SS, Chow LQ, Hwu W-J, Topalian SL, Hwu P, et al. Safety and activity of anti–Pd-L1 antibody in patients with advanced cancer. New Engl J Med (2012) 366(26):2455–65. doi: 10.1056/NEJMoa1200694
12. Reck M, Rodríguez-Abreu D, Robinson AG, Hui R, Csőszi T, Fülöp A, et al. Pembrolizumab versus chemotherapy for Pd-L1–positive non–Small-Cell lung cancer. N Engl J Med (2016) 375:1823–33. doi: 10.1056/NEJMoa1606774
13. Zhang Z, Liu X, Chen D, Yu J. Radiotherapy combined with immunotherapy: The dawn of cancer treatment. Signal Transduction Targeted Ther (2022) 7(1):1–34. doi: 10.1038/s41392-022-01102-y
14. Hui R, Özgüroğlu M, Villegas A, Daniel D, Vicente D, Murakami S, et al. Patient-reported outcomes with durvalumab after chemoradiotherapy in stage iii, unresectable non-Small-Cell lung cancer (Pacific): A randomised, controlled, phase 3 study. Lancet Oncol (2019) 20(12):1670–80. doi: 10.1016/S1470-2045(19)30519-4
15. Jabbour SK, Lee KH, Frost N, Breder V, Kowalski DM, Pollock T, et al. Pembrolizumab plus concurrent chemoradiation therapy in patients with unresectable, locally advanced, stage iii non–small cell lung cancer: The phase 2 keynote-799 nonrandomized trial. JAMA Oncol (2021) 7(9):1351–9. doi: 10.1001/jamaoncol.2021.2301
16. Antonia SJ, Villegas A, Daniel D, Vicente D, Murakami S, Hui R, et al. Durvalumab after chemoradiotherapy in stage iii non–Small-Cell lung cancer. New Engl J Med (2017) 377(20):1919–29. doi: 10.1056/NEJMoa1709937
17. Antonia SJ, Villegas A, Daniel D, Vicente D, Murakami S, Hui R, et al. Overall survival with durvalumab after chemoradiotherapy in stage iii nsclc. New Engl J Med (2018) 379(24):2342–50. doi: 10.1056/NEJMoa1809697
18. Kang J, Demaria S, Formenti S. Current clinical trials testing the combination of immunotherapy with radiotherapy. J immunotherapy Cancer (2016) 4(1):1–20. doi: 10.1186/s40425-016-0156-7
19. Spigel DR, Faivre-Finn C, Gray JE, Vicente D, Planchard D, Paz-Ares L, et al. Five-year survival outcomes from the pacific trial: Durvalumab after chemoradiotherapy in stage iii non–Small-Cell lung cancer. J Clin Oncol (2022) 40(12):1301. doi: 10.1200/JCO.21.01308
20. Gomez DR, Blumenschein GR Jr., Lee JJ, Hernandez M, Ye R, Camidge DR, et al. Local consolidative therapy versus maintenance therapy or observation for patients with oligometastatic non-Small-Cell lung cancer without progression after first-line systemic therapy: A multicentre, randomised, controlled, phase 2 study. Lancet Oncol (2016) 17(12):1672–82. doi: 10.1016/S1470-2045(16)30532-0
21. Iyengar P, Wardak Z, Gerber DE, Tumati V, Ahn C, Hughes RS, et al. Consolidative radiotherapy for limited metastatic non–Small-Cell lung cancer: A phase 2 randomized clinical trial. JAMA Oncol (2018) 4(1):e173501–e. doi: 10.1001/jamaoncol.2017.3501
22. Ball D, Mai GT, Vinod S, Babington S, Ruben J, Kron T, et al. Stereotactic ablative radiotherapy versus standard radiotherapy in stage 1 non-Small-Cell lung cancer (Trog 09.02 chisel): A phase 3, open-label, randomised controlled trial. Lancet Oncol (2019) 20(4):494–503. doi: 10.1016/S1470-2045(18)30896-9
23. Formenti SC. Optimizing dose per fraction: A new chapter in the story of the abscopal effect? Int J Radiat Oncology Biology Phys (2017) 99(3):677–9. doi: 10.1016/j.ijrobp.2017.07.028
24. Vanpouille-Box C, Alard A, Aryankalayil MJ, Sarfraz Y, Diamond JM, Schneider RJ, et al. DNA Exonuclease Trex1 regulates radiotherapy-induced tumour immunogenicity. Nat Commun (2017) 8(1):1–15. doi: 10.1038/ncomms15618
25. Lan J, Li R, Yin L-M, Deng L, Gui J, Chen B-Q, et al. Targeting myeloid-derived suppressor cells and programmed death ligand 1 confers therapeutic advantage of ablative hypofractionated radiation therapy compared with conventional fractionated radiation therapy. Int J Radiat Oncol Biol Phys (2018) 101(1):74–87. doi: 10.1016/j.ijrobp.2018.01.071
26. Chen Y, Gao M, Huang Z, Yu J, Meng X. Sbrt combined with pd-1/Pd-L1 inhibitors in nsclc treatment: A focus on the mechanisms, advances, and future challenges. J Hematol Oncol (2020) 13(1):1–17. doi: 10.1186/s13045-020-00940-z
27. Luke JJ, Lemons JM, Karrison TG, Pitroda SP, Melotek JM, Zha Y, et al. Safety and clinical activity of pembrolizumab and multisite stereotactic body radiotherapy in patients with advanced solid tumors. J Clin Oncol (2018) 36(16):1611. doi: 10.1200/JCO.2017.76.2229
28. Patel JD, Bestvina CM, Karrison T, Jelinek MJ, Juloori A, Pointer K, et al. Randomized phase I trial to evaluate c oncurrent or s equential I pilimumab, n ivolumab, and stereotactic body r adiotherapy in patients with stage iv non-small cell lung cancer (Cosinr study). Am Soc Clin Oncol (2020) 2020: 9616–9616. doi: 10.1200/JCO.2020.38.15_suppl.9616
29. Theelen WS, Chen D, Verma V, Hobbs BP, Peulen HM, Aerts JG, et al. Pembrolizumab with or without radiotherapy for metastatic non-Small-Cell lung cancer: A pooled analysis of two randomised trials. Lancet Respir Med (2021) 9(5):467–75. doi: 10.1016/S2213-2600(20)30391-X
30. Maity A, Mick R, Huang AC, George SM, Farwell MD, Lukens JN, et al. A phase I trial of pembrolizumab with hypofractionated radiotherapy in patients with metastatic solid tumours. Br J Cancer (2018) 119(10):1200–7. doi: 10.1038/s41416-018-0281-9
31. Welsh J, Menon H, Chen D, Verma V, Tang C, Altan M, et al. Pembrolizumab with or without radiation therapy for metastatic non-small cell lung cancer: A randomized phase I/Ii trial. J immunotherapy Cancer (2020) 8(2):e001001. doi: 10.1136/jitc-2020-001001
32. McBride S, Sherman E, Tsai CJ, Baxi S, Aghalar J, Eng J, et al. Randomized phase ii trial of nivolumab with stereotactic body radiotherapy versus nivolumab alone in metastatic head and neck squamous cell carcinoma. J Clin Oncol (2021) 39(1):30. doi: 10.1200/JCO.20.00290
33. Lin L, Kane N, Kobayashi N, Kono EA, Yamashiro JM, Nickols NG, et al. High-dose per fraction radiotherapy induces both antitumor immunity and immunosuppressive responses in prostate tumorshigh-dose radiation-induced immunity in prostate cancer. Clin Cancer Res (2021) 27(5):1505–15. doi: 10.1158/1078-0432.CCR-20-2293
34. Li A, Barsoumian HB, Schoenhals JE, Caetano MS, Wang X, Menon H, et al. Ido1 inhibition overcomes radiation-induced “Rebound immune suppression” by reducing numbers of Ido1-expressing myeloid-derived suppressor cells in the tumor microenvironment. Int J Radiat Oncol Biol Phys (2019) 104(4):903–12. doi: 10.1016/j.ijrobp.2019.03.022
35. Herrera FG, Romero P, Coukos G. Lighting up the tumor fire with low-dose irradiation. Trends Immunol (2022) 43(3):173–9. doi: 10.1016/j.it.2022.01.006
36. De Palma M, Coukos G, Hanahan D. A new twist on radiation oncology: Low-dose irradiation elicits immunostimulatory macrophages that unlock barriers to tumor immunotherapy. Cancer Cell (2013) 24(5):559–61. doi: 10.1016/j.ccr.2013.10.019
37. Lu K, He C, Guo N, Chan C, Ni K, Lan G, et al. Low-dose X-ray radiotherapy–radiodynamic therapy Via nanoscale metal–organic frameworks enhances checkpoint blockade immunotherapy. Nat Biomed Eng (2018) 2(8):600–10. doi: 10.1038/s41551-018-0203-4
38. He K, Barsoumian HB, Bertolet G, Verma V, Leuschner C, Koay EJ, et al. Novel use of low-dose radiotherapy to modulate the tumor microenvironment of liver metastases. Front Immunol (2021) 12. doi: 10.3389/fimmu.2021.812210
39. Savage T, Pandey S, Guha C. Postablation modulation after single high-dose radiation therapy improves tumor control Via enhanced immunomodulationimmunologic consequences of radiation fractionation. Clin Cancer Res (2020) 26(4):910–21. doi: 10.1158/1078-0432.CCR-18-3518
40. Barsoumian HB, Sezen D, Menon H, Younes AI, Hu Y, He K, et al. High plus low dose radiation strategy in combination with tigit and Pd1 blockade to promote systemic antitumor responses. Cancers (2022) 14(1):221. doi: 10.3390/cancers14010221
41. Barsoumian HB, Ramapriyan R, Younes AI, Caetano MS, Menon H, Comeaux NI, et al. Low-dose radiation treatment enhances systemic antitumor immune responses by overcoming the inhibitory stroma. J immunotherapy Cancer (2020) 8(2):e000537. doi: 10.1136/jitc-2020-000537
42. Yin L, Xue J, Li R, Zhou L, Deng L, Chen L, et al. Effect of low-dose radiation therapy on abscopal responses to hypofractionated radiation therapy and anti-Pd1 in mice and patients with non-small cell lung cancer. Int J Radiat Oncol Biol Phys (2020) 108(1):212–24. doi: 10.1016/j.ijrobp.2020.05.002
43. Menon H, Chen D, Ramapriyan R, Verma V, Barsoumian HB, Cushman TR, et al. Influence of low-dose radiation on abscopal responses in patients receiving high-dose radiation and immunotherapy. J immunotherapy Cancer (2019) 7(1):1–9. doi: 10.1186/s40425-019-0718-6
44. Sezen D, Patel RR, Tang C, Onstad M, Nagarajan P, Patel SP, et al. Immunotherapy combined with high-and low-dose radiation to all sites leads to complete clearance of disease in a patient with metastatic vaginal melanoma. Gynecologic Oncol (2021) 161(3):645–52. doi: 10.1016/j.ygyno.2021.03.017
45. Lederman M. The early history of radiotherapy: 1895–1939. Int J Radiat Oncol Biol Phys (1981) 7(5):639–48. doi: 10.1016/0360-3016(81)90379-5
46. Regaud C, Nogier T. Sterilization rontgenienne totale et definitive, sans radiodermite, des testicules Du belier adulte: Conditions de sa realisation. Compt Rend Soc Biol (1911) 70:202–3.
47. Jeraj R, Mackie TR, Balog J, Olivera G, Pearson D, Kapatoes J, et al. Radiation characteristics of helical tomotherapy. Med Phys (2004) 31(2):396–404. doi: 10.1118/1.1639148
48. Welsh JS, Patel RR, Ritter MA, Harari PM, Mackie TR, Mehta MP. Helical tomotherapy: An innovative technology and approach to radiation therapy. Technol Cancer Res Treat (2002) 1(4):311–6. doi: 10.1177/153303460200100413
49. Bortfeld T. Imrt: A review and preview. Phys Med Biol (2006) 51(13):R363. doi: 10.1088/0031-9155/51/13/R21
50. Girdhani S, Sachs R, Hlatky L. Biological effects of proton radiation: What we know and don’t know. Radiat Res (2013) 179(3):257–72. doi: 10.1667/RR2839.1
51. Pan HY, Jiang J, Hoffman KE, Tang C, Choi SL, Nguyen Q-N, et al. Comparative toxicities and cost of intensity-modulated radiotherapy, proton radiation, and stereotactic body radiotherapy among younger men with prostate cancer. J Clin Oncol (2018) 36(18):1823. doi: 10.1200/JCO.2017.75.5371
52. Bourhis J, Montay-Gruel P, Jorge PG, Bailat C, Petit B, Ollivier J, et al. Clinical translation of flash radiotherapy: Why and how? Radiotherapy Oncol (2019) 139:11–7. doi: 10.1016/j.radonc.2019.04.008
53. Bradley MO, Kohn KW. X-Ray induced DNA double strand break production and repair in mammalian cells as measured by neutral filter elution. Nucleic Acids Res (1979) 7(3):793–804. doi: 10.1093/nar/7.3.793
54. Toulany M. Targeting DNA double-strand break repair pathways to improve radiotherapy response. Genes (2019) 10(1):25. doi: 10.3390/genes10010025
55. Wang J-s, Wang H-j, Qian H-l. Biological effects of radiation on cancer cells. Military Med Res (2018) 5(1):1–10. doi: 10.1186/s40779-018-0167-4
56. Meijer TW, Kaanders JH, Span PN, Bussink J. Targeting hypoxia, hif-1, and tumor glucose metabolism to improve radiotherapy efficacy. Clin Cancer Res (2012) 18(20):5585–94. doi: 10.1158/1078-0432.CCR-12-0858
57. Wang Y, Liu Z-G, Yuan H, Deng W, Li J, Huang Y, et al. The reciprocity between radiotherapy and cancer immunotherapyradiosensitizing immunotherapy. Clin Cancer Res (2019) 25(6):1709–17. doi: 10.1158/1078-0432.CCR-18-2581
58. Victor T-S, Rech AJ, Maity A, Rengan R, Pauken KE, Stelekati E, et al. Radiation and dual checkpoint blockade activate non-redundant immune mechanisms in cancer. Nature (2015) 520(7547):373–7. doi: 10.1038/nature14292
59. Barker HE, Paget JT, Khan AA, Harrington KJ. The tumour microenvironment after radiotherapy: Mechanisms of resistance and recurrence. Nat Rev Cancer (2015) 15(7):409–25. doi: 10.1038/nrc3958
60. Weichselbaum RR, Liang H, Deng L, Fu Y-X. Radiotherapy and immunotherapy: A beneficial liaison? Nat Rev Clin Oncol (2017) 14(6):365–79. doi: 10.1038/nrclinonc.2016.211
61. Chen DS, Mellman I. Elements of cancer immunity and the cancer–immune set point. Nature (2017) 541(7637):321–30. doi: 10.1038/nature21349
62. Srinivas US, Tan BW, Vellayappan BA, Jeyasekharan AD. Ros and the DNA damage response in cancer. Redox Biol (2019) 25:101084. doi: 10.1016/j.redox.2018.101084
63. Jiang M, Chen P, Wang L, Li W, Chen B, Liu Y, et al. Cgas-sting, an important pathway in cancer immunotherapy. J Hematol Oncol (2020) 13(1):1–11. doi: 10.1186/s13045-020-00916-z
64. Chen Q, Sun L, Chen ZJ. Regulation and function of the cgas–sting pathway of cytosolic DNA sensing. Nat Immunol (2016) 17(10):1142–9. doi: 10.1038/ni.3558
65. Mackenzie KJ, Carroll P, Martin C-A, Murina O, Fluteau A, Simpson DJ, et al. Cgas surveillance of micronuclei links genome instability to innate immunity. Nature (2017) 548(7668):461–5. doi: 10.1038/nature23449
66. Deng L, Liang H, Xu M, Yang X, Burnette B, Arina A, et al. Sting-dependent cytosolic DNA sensing promotes radiation-induced type I interferon-dependent antitumor immunity in immunogenic tumors. Immunity (2014) 41(5):843–52. doi: 10.1016/j.immuni.2014.10.019
67. Wenzel J, Bekisch B, Uerlich M, Haller O, Bieber T, Tüting T. Type I interferon–associated recruitment of cytotoxic lymphocytes: A common mechanism in regressive melanocytic lesions. Am J Clin Pathol (2005) 124(1):37–48. doi: 10.1309/4EJ9KL7CGDENVVLE
68. Harlin H, Meng Y, Peterson AC, Zha Y, Tretiakova M, Slingluff C, et al. Chemokine expression in melanoma metastases associated with Cd8+ T-cell recruitment. Cancer Res (2009) 69(7):3077–85. doi: 10.1158/0008-5472.CAN-08-2281
69. Woo S-R, Corrales L, Gajewski TF. The sting pathway and the T cell-inflamed tumor microenvironment. Trends Immunol (2015) 36(4):250–6. doi: 10.1016/j.it.2015.02.003
70. Golden EB, Marciscano AE, Formenti SC. Radiation therapy and the in situ vaccination approach. Int J Radiat Oncol Biol Phys (2020) 108(4):891–8. doi: 10.1016/j.ijrobp.2020.08.023
71. Mouw KW, Goldberg MS, Konstantinopoulos PA, D’Andrea AD. DNA Damage and repair biomarkers of immunotherapy responsedna repair biomarkers of immunotherapy response. Cancer Discovery (2017) 7(7):675–93. doi: 10.1158/2159-8290.CD-17-0226
72. Galluzzi L, Vitale I, Warren S, Adjemian S, Agostinis P, Martinez AB, et al. Consensus guidelines for the definition, detection and interpretation of immunogenic cell death. J immunotherapy Cancer (2020) 8(1):e000337. doi: 10.1136/jitc-2019-000337corr1
73. Ashrafizadeh M, Farhood B, Musa AE, Taeb S, Najafi M. Damage-associated molecular patterns in tumor radiotherapy. Int Immunopharmacol (2020) 86:106761. doi: 10.1016/j.intimp.2020.106761
74. Gardai SJ, McPhillips KA, Frasch SC, Janssen WJ, Starefeldt A, Murphy-Ullrich JE, et al. Cell-surface calreticulin initiates clearance of viable or apoptotic cells through trans-activation of lrp on the phagocyte. Cell (2005) 123(2):321–34. doi: 10.1016/j.cell.2005.08.032
75. Serrano-del Valle A, Anel A, Naval J, Marzo I. Immunogenic cell death and immunotherapy of multiple myeloma. Front Cell Dev Biol (2019) 7:50. doi: 10.3389/fcell.2019.00050
76. Obeid M, Panaretakis T, Joza N, Tufi R, Tesniere A, Van Endert P, et al. Calreticulin exposure is required for the immunogenicity of Γ-irradiation and uvc light-induced apoptosis. Cell Death Differentiation (2007) 14(10):1848–50. doi: 10.1038/sj.cdd.4402201
77. Wang Y-J, Fletcher R, Yu J, Zhang L. Immunogenic effects of chemotherapy-induced tumor cell death. Genes Dis (2018) 5(3):194–203. doi: 10.1016/j.gendis.2018.05.003
78. Vermeer DW, Spanos WC, Vermeer PD, Bruns AM, Lee KM, Lee JH. Radiation-induced loss of cell surface Cd47 enhances immune-mediated clearance of human papillomavirus-positive cancer. Int J Cancer (2013) 133(1):120–9. doi: 10.1002/ijc.28015
79. Matlung HL, Szilagyi K, Barclay NA, van den Berg TK. The Cd47-sirpα signaling axis as an innate immune checkpoint in cancer. Immunol Rev (2017) 276(1):145–64. doi: 10.1111/imr.12527
80. Candas-Green D, Xie B, Huang J, Fan M, Wang A, Menaa C, et al. Dual blockade of Cd47 and Her2 eliminates radioresistant breast cancer cells. Nat Commun (2020) 11(1):1–15. doi: 10.1038/s41467-020-18245-7
81. Veillette A, Chen J. Sirpα–Cd47 immune checkpoint blockade in anticancer therapy. Trends Immunol (2018) 39(3):173–84. doi: 10.1016/j.it.2017.12.005
82. Chao MP, Weissman IL, Majeti R. The Cd47–sirpα pathway in cancer immune evasion and potential therapeutic implications. Curr Opin Immunol (2012) 24(2):225–32. doi: 10.1016/j.coi.2012.01.010
83. Huang C-Y, Ye Z-H, Huang M-Y, Lu J-J. Regulation of Cd47 expression in cancer cells. Trans Oncol (2020) 13(12):100862. doi: 10.1016/j.tranon.2020.100862
84. Feng R, Zhao H, Xu J, Shen C. Cd47: The next checkpoint target for cancer immunotherapy. Crit Rev Oncology/Hematology (2020) 152:103014. doi: 10.1016/j.critrevonc.2020.103014
85. Apetoh L, Ghiringhelli F, Tesniere A, Obeid M, Ortiz C, Criollo A, et al. Toll-like receptor 4–dependent contribution of the immune system to anticancer chemotherapy and radiotherapy. Nat Med (2007) 13(9):1050–9. doi: 10.1038/nm1622
86. Rapoport BL, Anderson R. Realizing the clinical potential of immunogenic cell death in cancer chemotherapy and radiotherapy. Int J Mol Sci (2019) 20(4):959. doi: 10.3390/ijms20040959
87. Wu C-Y, Yang L-H, Yang H-Y, Knoff J, Peng S, Lin Y-H, et al. Enhanced cancer radiotherapy through immunosuppressive stromal cell destruction in tumorscancer radiotherapy enhanced by stromal cell destruction. Clin Cancer Res (2014) 20(3):644–57. doi: 10.1158/1078-0432.CCR-13-1334
88. Golden EB, Apetoh L. Radiotherapy and immunogenic cell death. Semin Radiat Oncol (2015) 25(1):11–17. doi: 10.1016/j.semradonc.2014.07.005
89. Zitvogel L, Galluzzi L, Kepp O, Smyth MJ, Kroemer G. Type I interferons in anticancer immunity. Nat Rev Immunol (2015) 15(7):405–14. doi: 10.1038/nri3845
90. Pasi F, Paolini A, Nano R, Di Liberto R, Capelli E. Effects of single or combined treatments with radiation and chemotherapy on survival and danger signals expression in glioblastoma cell lines. BioMed Res Int (2014) 2014:453497. doi: 10.1155/2014/453497
91. Binder R, Vatner R, Srivastava P. The heat-shock protein receptors: Some answers and more questions. Tissue Antigens (2004) 64(4):442–51. doi: 10.1111/j.1399-0039.2004.00299.x
92. Mole R. Whole body irradiation–radiobiology or medicine? Br J Radiol (1953) 26(305):234–41. doi: 10.1259/0007-1285-26-305-234
93. Liu Y, Dong Y, Kong L, Shi F, Zhu H, Yu J. Abscopal effect of radiotherapy combined with immune checkpoint inhibitors. J Hematol Oncol (2018) 11(1):1–15. doi: 10.1186/s13045-018-0647-8
94. Ngwa W, Irabor OC, Schoenfeld JD, Hesser J, Demaria S, Formenti SC. Using immunotherapy to boost the abscopal effect. Nat Rev Cancer (2018) 18(5):313–22. doi: 10.1038/nrc.2018.6
95. Reynders K, Illidge T, Siva S, Chang JY, De Ruysscher D. The abscopal effect of local radiotherapy: Using immunotherapy to make a rare event clinically relevant. Cancer Treat Rev (2015) 41(6):503–10. doi: 10.1016/j.ctrv.2015.03.011
96. Rodríguez-Ruiz ME, Vanpouille-Box C, Melero I, Formenti SC, Demaria S. Immunological mechanisms responsible for radiation-induced abscopal effect. Trends Immunol (2018) 39(8):644–55. doi: 10.1016/j.it.2018.06.001
97. Buchwald ZS, Wynne J, Nasti TH, Zhu S, Mourad WF, Yan W, et al. Radiation, immune checkpoint blockade and the abscopal effect: A critical review on timing, dose and fractionation. Front Oncol (2018) 8:612. doi: 10.3389/fonc.2018.00612
98. Hanahan D, Weinberg RA. Hallmarks of cancer: The next generation. Cell (2011) 144(5):646–74. doi: 10.1016/j.cell.2011.02.013
99. Donlon N, Power R, Hayes C, Reynolds J, Lysaght J. Radiotherapy, immunotherapy, and the tumour microenvironment: Turning an immunosuppressive milieu into a therapeutic opportunity. Cancer Lett (2021) 502:84–96. doi: 10.1016/j.canlet.2020.12.045
100. Riaz N, Havel JJ, Makarov V, Desrichard A, Urba WJ, Sims JS, et al. Tumor and microenvironment evolution during immunotherapy with nivolumab. Cell (2017) 171(4):934–49.e16. doi: 10.1016/j.cell.2017.09.028
101. Gajewski TF, Schreiber H, Fu Y-X. Innate and adaptive immune cells in the tumor microenvironment. Nat Immunol (2013) 14(10):1014–22. doi: 10.1038/ni.2703
102. Junttila MR, De Sauvage FJ. Influence of tumour micro-environment heterogeneity on therapeutic response. Nature (2013) 501(7467):346–54. doi: 10.1038/nature12626
103. O’Donnell JS, Teng MW, Smyth MJ. Cancer immunoediting and resistance to T cell-based immunotherapy. Nat Rev Clin Oncol (2019) 16(3):151–67. doi: 10.1038/s41571-018-0142-8
104. Herbst RS, Soria J-C, Kowanetz M, Fine GD, Hamid O, Gordon MS, et al. Predictive correlates of response to the anti-Pd-L1 antibody Mpdl3280a in cancer patients. Nature (2014) 515(7528):563–7. doi: 10.1038/nature14011
105. Rosenberg JE, Hoffman-Censits J, Powles T, van der Heijden MS, Balar AV, Necchi A, et al. Atezolizumab in patients with locally advanced and metastatic urothelial carcinoma who have progressed following treatment with platinum-based chemotherapy: A single-arm, multicentre, phase 2 trial. Lancet (2016) 387(10031):1909–20. doi: 10.1016/S0140-6736(16)00561-4
106. Garon EB, Rizvi NA, Hui R, Leighl N, Balmanoukian AS, Eder JP, et al. Pembrolizumab for the treatment of non–Small-Cell lung cancer. New Engl J Med (2015) 372(21):2018–28. doi: 10.1056/NEJMoa1501824
107. Joyce JA, Fearon DT. T Cell exclusion, immune privilege, and the tumor microenvironment. Science (2015) 348(6230):74–80. doi: 10.1126/science.aaa6204
108. Vanpouille-Box C, Formenti SC. Dual transforming growth factor-B and programmed death-1 blockade: A strategy for immune-excluded tumors? Trends Immunol (2018) 39(6):435–7. doi: 10.1016/j.it.2018.03.002
109. Nikolos F, Hayashi K, Hoi XP, Alonzo ME, Mo Q, Kasabyan A, et al. Cell death-induced immunogenicity enhances chemoimmunotherapeutic response by converting immune-excluded into T-cell inflamed bladder tumors. Nat Commun (2022) 13(1):1–16. doi: 10.1038/s41467-022-29026-9
110. Frey B, Rubner Y, Wunderlich R, Weiss E-M, Pockley AG, Fietkau R, et al. Induction of abscopal anti-tumor immunity and immunogenic tumor cell death by ionizing irradiation-implications for cancer therapies. Curr medicinal Chem (2012) 19(12):1751–64. doi: 10.2174/092986712800099811
111. Formenti SC, Demaria S. Combining radiotherapy and cancer immunotherapy: A paradigm shift. JNCI: J Natl Cancer Institute (2013) 105(4):256–65. doi: 10.1093/jnci/djs629
112. Park B, Yee C, Lee K-M. The effect of radiation on the immune response to cancers. Int J Mol Sci (2014) 15(1):927–43. doi: 10.3390/ijms15010927
113. Walle T, Martinez Monge R, Cerwenka A, Ajona D, Melero I, Lecanda F. Radiation effects on antitumor immune responses: Current perspectives and challenges. Ther Adv Med Oncol (2018) 10:1758834017742575. doi: 10.1177/1758834017742575
114. Rodriguez-Ruiz ME, Rodriguez I, Garasa S, Barbes B, Solorzano JL, Perez-Gracia JL, et al. Abscopal effects of radiotherapy are enhanced by combined immunostimulatory mabs and are dependent on Cd8 T cells and crossprimingradioimmunotherapy and abscopal effects. Cancer Res (2016) 76(20):5994–6005. doi: 10.1158/0008-5472.CAN-16-0549
115. Taieb J, Chaput N, Ménard C, Apetoh L, Ullrich E, Bonmort M, et al. A novel dendritic cell subset involved in tumor immunosurveillance. Nat Med (2006) 12(2):214–9. doi: 10.1038/nm1356
116. Lee Y, Auh SL, Wang Y, Burnette B, Wang Y, Meng Y, et al. Therapeutic effects of ablative radiation on local tumor require Cd8+ T cells: Changing strategies for cancer treatment. Blood J Am Soc Hematol (2009) 114(3):589–95. doi: 10.1182/blood-2009-02-206870
117. Sharabi AB, Nirschl CJ, Kochel CM, Nirschl TR, Francica BJ, Velarde E, et al. Stereotactic radiation therapy augments antigen-specific Pd-1–mediated antitumor immune responses Via cross-presentation of tumor antigencross-presentation of tumor antigens augmented by radiotherapy. Cancer Immunol Res (2015) 3(4):345–55. doi: 10.1158/2326-6066.CIR-14-0196
118. Gupta A, Probst HC, Vuong V, Landshammer A, Muth S, Yagita H, et al. Radiotherapy promotes tumor-specific effector Cd8+ T cells Via dendritic cell activation. J Immunol (2012) 189(2):558–66. doi: 10.4049/jimmunol.1200563
119. Vonderheide RH, Glennie MJ. Agonistic Cd40 antibodies and cancer therapy. Clin Cancer Res (2013) 19(5):1035–43. doi: 10.1158/1078-0432.CCR-12-2064
120. Reits EA, Hodge JW, Herberts CA, Groothuis TA, Chakraborty M, K. Wansley E, et al. Radiation modulates the peptide repertoire, enhances mhc class I expression, and induces successful antitumor immunotherapy. J Exp Med (2006) 203(5):1259–71. doi: 10.1084/jem.20052494
121. Lugade AA, Sorensen EW, Gerber SA, Moran JP, Frelinger JG, Lord EM. Radiation-induced ifn-Γ production within the tumor microenvironment influences antitumor immunity. J Immunol (2008) 180(5):3132–9. doi: 10.4049/jimmunol.180.5.3132
122. Kim J-Y, Son Y-O, Park S-W, Bae J-H, Chung JS, Kim HH, et al. Increase of Nkg2d ligands and sensitivity to nk cell-mediated cytotoxicity of tumor cells by heat shock and ionizing radiation. Exp Mol Med (2006) 38(5):474–84. doi: 10.1038/emm.2006.56
123. Vatner RE, Formenti SC. Myeloid-derived cells in tumors: Effects of radiation. Semin Radiat Oncol (2015) 25(1):18–27. doi: 10.1016/j.semradonc.2014.07.008
124. Matsumura S, Wang B, Kawashima N, Braunstein S, Badura M, Cameron TO, et al. Radiation-induced Cxcl16 release by breast cancer cells attracts effector T cells. J Immunol (2008) 181(5):3099–107. doi: 10.4049/jimmunol.181.5.3099
125. Meng Y, Mauceri HJ, Khodarev NN, Darga TE, Pitroda SP, Beckett MA, et al. Ad. Egr-tnf and local ionizing radiation suppress metastases by interferon-B-Dependent activation of antigen-specific Cd8+ T cells. Mol Ther (2010) 18(5):912–20. doi: 10.1038/mt.2010.18
126. Lanitis E, Irving M, Coukos G. Targeting the tumor vasculature to enhance T cell activity. Curr Opin Immunol (2015) 33:55–63. doi: 10.1016/j.coi.2015.01.011
127. Fridman WH, Sautès-Fridman C, Galon J. The immune contexture in human tumours: Impact on clinical outcome. Nat Rev Cancer (2012) 12(4):298–306. doi: 10.1038/nrc3245
128. Dushyanthen S, Beavis PA, Savas P, Teo ZL, Zhou C, Mansour M, et al. Relevance of tumor-infiltrating lymphocytes in breast cancer. BMC Med (2015) 13(1):1–13. doi: 10.1186/s12916-015-0431-3
129. Anitei M-G, Zeitoun G, Mlecnik B, Marliot F, Haicheur N, Todosi A-M, et al. Prognostic and predictive values of the immunoscore in patients with rectal cancer. Clin Cancer Res (2014) 20(7):1891–9. doi: 10.1158/1078-0432.CCR-13-2830
130. Kachikwu EL, Iwamoto KS, Liao Y-P, DeMarco JJ, Agazaryan N, Economou JS, et al. Radiation enhances regulatory T cell representation. Int J Radiat Oncol Biol Phys (2011) 81(4):1128–35. doi: 10.1016/j.ijrobp.2010.09.034
131. Brandmaier A, Formenti SC. The impact of radiation therapy on innate and adaptive tumor immunity. Semin Radiat Oncol (2020) 30(2):139–44. doi: 10.1016/j.semradonc.2019.12.005
132. Facciabene A, Motz GT, Coukos G. T-Regulatory cells: Key players in tumor immune escape and angiogenesis. Cancer Res (2012) 72(9):2162–71. doi: 10.1158/0008-5472.CAN-11-3687
133. Fadul CE, Fisher JL, Hampton TH, Lallana EC, Li Z, Gui J, et al. Immune response in patients with newly diagnosed glioblastoma multiforme treated with intranodal autologous tumor lysate-dendritic cell vaccination after radiation chemotherapy. J immunotherapy (Hagerstown Md: 1997) (2011) 34(4):382. doi: 10.1097/CJI.0b013e318215e300
134. Schuler PJ, Harasymczuk M, Schilling B, Saze Z, Strauss L, Lang S, et al. Effects of adjuvant chemoradiotherapy on the frequency and function of regulatory T cells in patients with head and neck Cancercd4+ Cd39+ treg and crt. Clin Cancer Res (2013) 19(23):6585–96. doi: 10.1158/1078-0432.CCR-13-0900
135. Qinfeng S, Depu W, Xiaofeng Y, Shah W, Hongwei C, Yili W. In situ observation of the effects of local irradiation on cytotoxic and regulatory T lymphocytes in cervical cancer tissue. Radiat Res (2013) 179(5):584–9. doi: 10.1667/RR3155.1
136. Ji D, Song C, Li Y, Xia J, Wu Y, Jia J, et al. Combination of radiotherapy and suppression of tregs enhances abscopal antitumor effect and inhibits metastasis in rectal cancer. J immunotherapy Cancer (2020) 8(2):e000826. doi: 10.1136/jitc-2020-000826
137. Zitvogel L, Kroemer G. Subversion of anticancer immunosurveillance by radiotherapy. Nat Immunol (2015) 16(10):1005–7. doi: 10.1038/ni.3236
138. de Streel G, Bertrand C, Chalon N, Liénart S, Bricard O, Lecomte S, et al. Selective inhibition of tgf-B1 produced by garp-expressing tregs overcomes resistance to pd-1/Pd-L1 blockade in cancer. Nat Commun (2020) 11(1):1–15. doi: 10.1038/s41467-020-17811-3
139. Kho VM, Mekers VE, Span PN, Bussink J, Adema GJ. Radiotherapy and Cgas/Sting signaling: Impact on mdscs in the tumor microenvironment. Cell Immunol (2021) 362:104298. doi: 10.1016/j.cellimm.2021.104298
140. Liang H, Deng L, Hou Y, Meng X, Huang X, Rao E, et al. Host sting-dependent mdsc mobilization drives extrinsic radiation resistance. Nat Commun (2017) 8(1):1–10. doi: 10.1038/s41467-017-01566-5
141. Ostrand-Rosenberg S. Myeloid-derived suppressor cells: More mechanisms for inhibiting antitumor immunity. Cancer immunology immunotherapy (2010) 59(10):1593–600. doi: 10.1007/s00262-010-0855-8
142. Nagaraj S, Gabrilovich DI. Myeloid-derived suppressor cells in human cancer. Cancer J (2010) 16(4):348–53. doi: 10.1097/PPO.0b013e3181eb3358
143. Seifert L, Werba G, Tiwari S, Ly NNG, Nguy S, Alothman S, et al. Radiation therapy induces macrophages to suppress T-cell responses against pancreatic tumors in mice. Gastroenterology (2016) 150(7):1659–72.e5. doi: 10.1053/j.gastro.2016.02.070
144. Xu J, Escamilla J, Mok S, David J, Priceman S, West B, et al. Csf1r signaling blockade stanches tumor-infiltrating myeloid cells and improves the efficacy of radiotherapy in prostate cancer. Cancer Res (2013) 73(9):2782–94. doi: 10.1158/0008-5472.CAN-12-3981
145. Mantovani A, Schioppa T, Porta C, Allavena P, Sica A. Role of tumor-associated macrophages in tumor progression and invasion. Cancer Metastasis Rev (2006) 25(3):315–22. doi: 10.1007/s10555-006-9001-7
146. Huang Y, Snuderl M, Jain RK. Polarization of tumor-associated macrophages: A novel strategy for vascular normalization and antitumor immunity. Cancer Cell (2011) 19(1):1–2. doi: 10.1016/j.ccr.2011.01.005
147. Allavena P, Sica A, Garlanda C, Mantovani A. The yin-yang of tumor-associated macrophages in neoplastic progression and immune surveillance. Immunol Rev (2008) 222(1):155–61. doi: 10.1111/j.1600-065X.2008.00607.x
148. Rodriguez PC, Quiceno DG, Zabaleta J, Ortiz B, Zea AH, Piazuelo MB, et al. Arginase I production in the tumor microenvironment by mature myeloid cells inhibits T-cell receptor expression and antigen-specific T-cell responses. Cancer Res (2004) 64(16):5839–49. doi: 10.1158/0008-5472.CAN-04-0465
149. Vanpouille-Box C, Diamond JM, Pilones KA, Zavadil J, Babb JS, Formenti SC, et al. Tgfβ is a master regulator of radiation therapy-induced antitumor immunity. Cancer Res (2015) 75(11):2232–42. doi: 10.1158/0008-5472.CAN-14-3511
150. Tuxhorn JA, McAlhany SJ, Yang F, Dang TD, Rowley DR. Inhibition of transforming growth factor-B activity decreases angiogenesis in a human prostate cancer-reactive stroma xenograft model. Cancer Res (2002) 62(21):6021–5.
151. Colton M, Cheadle EJ, Honeychurch J, Illidge TM. Reprogramming the tumour microenvironment by radiotherapy: Implications for radiotherapy and immunotherapy combinations. Radiat Oncol (2020) 15(1):1–11. doi: 10.1186/s13014-020-01678-1
152. Lim Y. Radiation-induced change of pd-1/Pd-L1 immune checkpoint in mouse colon cancer models. Ann Oncol (2019) 30:vii24. doi: 10.1093/annonc/mdz413.087
153. Illidge T, Lipowska-Bhalla G, Cheadle E, Honeychurch J, Poon E, Morrow M, et al. Radiation therapy induces an adaptive upregulation of pd-L1 on tumor cells which may limit the efficacy of the anti-tumor immune response but can be circumvented by anti-Pd-L1. Int J Radiat Oncology Biology Phys (2014) 90(1):S776. doi: 10.1016/j.ijrobp.2014.05.2247
154. Bezjak A, Paulus R, Gaspar LE, Timmerman RD, Straube WL, Ryan WF, et al. Safety and efficacy of a five-fraction stereotactic body radiotherapy schedule for centrally located non–Small-Cell lung cancer: Nrg Oncology/Rtog 0813 trial. J Clin Oncol (2019) 37(15):1316. doi: 10.1200/JCO.18.00622
155. Cheung P, Patel S, North SA, Sahgal A, Chu W, Soliman H, et al. Stereotactic radiotherapy for oligoprogression in metastatic renal cell cancer patients receiving tyrosine kinase inhibitor therapy: A phase 2 prospective multicenter study. Eur Urol (2021) 80(6):693–700. doi: 10.1016/j.eururo.2021.07.026
156. Pucar D, Hricak H, Shukla-Dave A, Kuroiwa K, Drobnjak M, Eastham J, et al. Clinically significant prostate cancer local recurrence after radiation therapy occurs at the site of primary tumor: Magnetic resonance imaging and step-section pathology evidence. Int J Radiat Oncol Biol Phys (2007) 69(1):62–9. doi: 10.1016/j.ijrobp.2007.03.065
157. Robert C. A decade of immune-checkpoint inhibitors in cancer therapy. Nat Commun (2020) 11(1):1–3. doi: 10.1038/s41467-020-17670-y
158. Brahmer JR, Pardoll DM. Immune checkpoint inhibitors: Making immunotherapy a reality for the treatment of lung cancer. Cancer Immunol Res (2013) 1(2):85–91. doi: 10.1158/2326-6066.CIR-13-0078
159. Fishman M. Challenges facing the development of cancer vaccines. Cancer Vaccines (2014) 1139:543–53. doi: 10.1007/978-1-4939-0345-0_39
160. Ribas A, Wolchok JD. Cancer immunotherapy using checkpoint blockade. Science (2018) 359(6382):1350–5. doi: 10.1126/science.aar4060
161. Sharma P, Hu-Lieskovan S, Wargo JA, Ribas A. Primary, adaptive, and acquired resistance to cancer immunotherapy. Cell (2017) 168(4):707–23. doi: 10.1016/j.cell.2017.01.017
162. Sharabi AB, Lim M, DeWeese TL, Drake CG. Radiation and checkpoint blockade immunotherapy: Radiosensitisation and potential mechanisms of synergy. Lancet Oncol (2015) 16(13):e498–509. doi: 10.1016/S1470-2045(15)00007-8
163. Hodge JW, Sharp HJ, Gameiro SR. Abscopal regression of antigen disparate tumors by antigen cascade after systemic tumor vaccination in combination with local tumor radiation. Cancer Biotherapy Radiopharmaceuticals (2012) 27(1):12–22. doi: 10.1089/cbr.2012.1202
164. Postow MA, Callahan MK, Barker CA, Yamada Y, Yuan J, Kitano S, et al. Immunologic correlates of the abscopal effect in a patient with melanoma. New Engl J Med (2012) 366(10):925–31. doi: 10.1056/NEJMoa1112824
165. Hiniker SM, Chen DS, Reddy S, Chang DT, Jones JC, Mollick JA, et al. A systemic complete response of metastatic melanoma to local radiation and immunotherapy. Trans Oncol (2012) 5(6):404–7. doi: 10.1593/tlo.12280
166. Golden EB, Demaria S, Schiff PB, Chachoua A, Formenti SC. An abscopal response to radiation and ipilimumab in a patient with metastatic non–small cell lung cancer. Cancer Immunol Res (2013) 1(6):365–72. doi: 10.1158/2326-6066.CIR-13-0115
167. Hiniker SM, Reddy SA, Maecker HT, Subrahmanyam PB, Rosenberg-Hasson Y, Swetter SM, et al. A prospective clinical trial combining radiation therapy with systemic immunotherapy in metastatic melanoma. Int J Radiat Oncol Biol Phys (2016) 96(3):578–88. doi: 10.1016/j.ijrobp.2016.07.005
168. Theelen WS, Peulen HM, Lalezari F, van der Noort V, De Vries JF, Aerts JG, et al. Effect of pembrolizumab after stereotactic body radiotherapy vs pembrolizumab alone on tumor response in patients with advanced non–small cell lung cancer: Results of the pembro-rt phase 2 randomized clinical trial. JAMA Oncol (2019) 5(9):1276–82. doi: 10.1001/jamaoncol.2019.1478
169. Bernstein MB, Krishnan S, Hodge JW, Chang JY. Immunotherapy and stereotactic ablative radiotherapy (Isabr): A curative approach? Nat Rev Clin Oncol (2016) 13(8):516–24. doi: 10.1038/nrclinonc.2016.30
170. Ashrafizadeh M, Farhood B, Musa AE, Taeb S, Rezaeyan A, Najafi M. Abscopal effect in radioimmunotherapy. Int Immunopharmacol (2020) 85:106663. doi: 10.1016/j.intimp.2020.106663
171. Morisada M, Clavijo PE, Moore E, Sun L, Chamberlin M, Van Waes C, et al. Pd-1 blockade reverses adaptive immune resistance induced by high-dose hypofractionated but not low-dose daily fractionated radiation. Oncoimmunology (2018) 7(3):e1395996. doi: 10.1080/2162402X.2017.1395996
172. Dewan MZ, Galloway AE, Kawashima N, Dewyngaert JK, Babb JS, Formenti SC, et al. Fractionated but not single-dose radiotherapy induces an immune-mediated abscopal effect when combined with anti–Ctla-4 antibodyfractionated radiation synergizes with immunotherapy. Clin Cancer Res (2009) 15(17):5379–88. doi: 10.1158/1078-0432.CCR-09-0265
173. Cai X, Chiu Y-H, Chen ZJ. The cgas-Cgamp-Sting pathway of cytosolic DNA sensing and signaling. Mol Cell (2014) 54(2):289–96. doi: 10.1016/j.molcel.2014.03.040
174. Muroyama Y, Nirschl TR, Kochel CM, Lopez-Bujanda Z, Theodros D, Mao W, et al. Stereotactic radiotherapy increases functionally suppressive regulatory T cells in the tumor microenvironmentexpansion of suppressive til-tregs after radiotherapy. Cancer Immunol Res (2017) 5(11):992–1004. doi: 10.1158/2326-6066.CIR-17-0040
175. Monjazeb AM, Giobbie-Hurder A, Lako A, Thrash EM, Brennick RC, Kao KZ, et al. A randomized trial of combined pd-L1 and ctla-4 inhibition with targeted low-dose or hypofractionated radiation for patients with metastatic colorectal cancerpd-L1/Ctla-4 inhibition with radiation for colorectal cancer. Clin Cancer Res (2021) 27(9):2470–80. doi: 10.1158/1078-0432.CCR-20-4632
176. Wang Y. Advances in hypofractionated irradiation-induced immunosuppression of tumor microenvironment. Front Immunol (2021) 11:612072. doi: 10.3389/fimmu.2020.612072
177. Begg K, Tavassoli M. Inside the hypoxic tumour: Reprogramming of the ddr and radioresistance. Cell Death Discovery (2020) 6(1):1–15. doi: 10.1038/s41420-020-00311-0
178. Mavragani IV, Laskaratou DA, Frey B, Candéias SM, Gaipl US, Lumniczky K, et al. Key mechanisms involved in ionizing radiation-induced systemic effects. a current review. Toxicol Res (2016) 5(1):12–33. doi: 10.1039/c5tx00222b
179. Klug F, Prakash H, Huber PE, Seibel T, Bender N, Halama N, et al. Low-dose irradiation programs macrophage differentiation to an Inos+/M1 phenotype that orchestrates effective T cell immunotherapy. Cancer Cell (2013) 24(5):589–602. doi: 10.1016/j.ccr.2013.09.014
180. Patel RR, He K, Barsoumian HB, Chang JY, Tang C, Verma V, et al. High-dose irradiation in combination with non-ablative low-dose radiation to treat metastatic disease after progression on immunotherapy: Results of a phase ii trial. Radiotherapy Oncol (2021) 162:60–7. doi: 10.1016/j.radonc.2021.06.037
181. Lin EY, Li J-F, Gnatovskiy L, Deng Y, Zhu L, Grzesik DA, et al. Macrophages regulate the angiogenic switch in a mouse model of breast cancer. Cancer Res (2006) 66(23):11238–46. doi: 10.1158/0008-5472.CAN-06-1278
182. Prakash H, Klug F, Nadella V, Mazumdar V, Schmitz-Winnenthal H, Umansky L. Low doses of gamma irradiation potentially modifies immunosuppressive tumor microenvironment by retuning tumor-associated macrophages: Lesson from insulinoma. Carcinogenesis (2016) 37(3):301–13. doi: 10.1093/carcin/bgw007
183. Lapeyre-Prost A, Terme M, Pernot S, Pointet A-L, Voron T, Tartour E, et al. Immunomodulatory activity of vegf in cancer. Int Rev Cell Mol Biol (2017) 330:295–342. doi: 10.1016/bs.ircmb.2016.09.007
184. Thomas AA, Fisher JL, Hampton TH, Christensen BC, Tsongalis GJ, Rahme GJ, et al. Immune modulation associated with vascular endothelial growth factor (Vegf) blockade in patients with glioblastoma. Cancer Immunology Immunotherapy (2017) 66(3):379–89. doi: 10.1007/s00262-016-1941-3
185. Voron T, Marcheteau E, Pernot S, Colussi O, Tartour E, Taieb J, et al. Control of the immune response by pro-angiogenic factors. Front Oncol (2014) 4:70. doi: 10.3389/fonc.2014.00070
186. Nadella V, Singh S, Jain A, Jain M, Vasquez KM, Sharma A, et al. Low dose radiation primed inos+ M1macrophages modulate angiogenic programming of tumor derived endothelium. Mol carcinogenesis (2018) 57(11):1664–71. doi: 10.1002/mc.22879
187. Yang G, Kong Q, Wang G, Jin H, Zhou L, Yu D, et al. Low-dose ionizing radiation induces direct activation of natural killer cells and provides a novel approach for adoptive cellular immunotherapy. Cancer Biotherapy Radiopharmaceuticals (2014) 29(10):428–34. doi: 10.1089/cbr.2014.1702
188. Sonn CH, Choi JR, Kim T-J, Yu Y-B, Kim K, Shin SC, et al. Augmentation of natural cytotoxicity by chronic low-dose ionizing radiation in murine natural killer cells primed by il-2. J Radiat Res (2012) 53(6):823–9. doi: 10.1093/jrr/rrs037
189. Cheda A, Wrembel-Wargocka J, Lisiak E, Nowosielska EM, Marciniak M, Janiak MK. Single low doses of X rays inhibit the development of experimental tumor metastases and trigger the activities of nk cells in mice. Radiat Res (2004) 161(3):335–40. doi: 10.1667/RR3123
190. Herrera FG, Ronet C, de Olza MO, Barras D, Crespo I, Andreatta M, et al. Low-dose radiotherapy reverses tumor immune desertification and resistance to immunotherapy. Cancer Discovery (2022) 12(1):108–33. doi: 10.1158/2159-8290.CD-21-0003
191. Zhou L, Zhang X, Li H, Niu C, Yu D, Yang G, et al. Validating the pivotal role of the immune system in low-dose radiation-induced tumor inhibition in Lewis lung cancer-bearing mice. Cancer Med (2018) 7(4):1338–48. doi: 10.1002/cam4.1344
192. Hashimoto S, Shirato H, Hosokawa M, Nishioka T, Kuramitsu Y, Matushita K, et al. The suppression of metastases and the change in host immune response after low-dose total-body irradiation in tumor-bearing rats. Radiat Res (1999) 151(6):717–24. doi: 10.2307/3580211
193. Sonanini D, Griessinger CM, Schörg BF, Knopf P, Dittmann K, Röcken M, et al. Low-dose total body irradiation facilitates antitumoral Th1 immune responses. Theranostics (2021) 11(16):7700. doi: 10.7150/thno.61459
194. Wang B, Li B, Dai Z, Ren S, Bai M, Wang Z, et al. Low-dose splenic radiation inhibits liver tumor development of rats through functional changes in Cd4+ Cd25+ treg cells. Int J Biochem Cell Biol (2014) 55:98–108. doi: 10.1016/j.biocel.2014.08.014
195. Liu R, Xiong S, Zhang L, Chu Y. Enhancement of antitumor immunity by low-dose total body irradiationis associated with selectively decreasing the proportion and number of T regulatorycells. Cell Mol Immunol (2010) 7(2):157–62. doi: 10.1038/cmi.2009.117
196. Nowosielska EM, Cheda A, Pociegiel M, Cheda L, Szymański P, Wiedlocha A. Effects of a unique combination of the whole-body low dose radiotherapy with inactivation of two immune checkpoints and/or a heat shock protein on the transplantable lung cancer in mice. Int J Mol Sci (2021) 22(12):6309. doi: 10.3390/ijms22126309
197. Patel RB, Hernandez R, Carlson P, Grudzinski J, Bates AM, Jagodinsky JC, et al. Low-dose targeted radionuclide therapy renders immunologically cold tumors responsive to immune checkpoint blockade. Sci Trans Med (2021) 13(602):eabb3631. doi: 10.1126/scitranslmed.abb3631
198. Schoenfeld JD, Giobbie-Hurder A, Ranasinghe S, Kao KZ, Lako A, Tsuji J, et al. Durvalumab plus tremelimumab alone or in combination with low-dose or hypofractionated radiotherapy in metastatic non-Small-Cell lung cancer refractory to previous pd (L)-1 therapy: An open-label, multicentre, randomised, phase 2 trial. Lancet Oncol (2022) 23(2):279–91. doi: 10.1016/S1470-2045(21)00658-6
199. Liu J, Zhou J, Wu M, Hu C, Yang J, Li D, et al. Low-dose total body irradiation can enhance systemic immune related response induced by hypo-fractionated radiation. Front Immunol (2019) 10:317. doi: 10.3389/fimmu.2019.00317
200. Welsh JW, Tang C, De Groot P, Naing A, Hess KR, Heymach JV, et al. Phase ii trial of ipilimumab with stereotactic radiation therapy for metastatic disease: Outcomes, toxicities, and low-dose radiation–related abscopal responsesipilimumab and sabr for lung or liver metastases. Cancer Immunol Res (2019) 7(12):1903–9. doi: 10.1158/2326-6066.CIR-18-0793
201. Easwaran H, Tsai H-C, Baylin SB. Cancer epigenetics: Tumor heterogeneity, plasticity of stem-like states, and drug resistance. Mol Cell (2014) 54(5):716–27. doi: 10.1016/j.molcel.2014.05.015
202. Marusyk A, Polyak K. Tumor heterogeneity: Causes and consequences. Biochim Biophys Acta (BBA)-Reviews Cancer (2010) 1805(1):105–17. doi: 10.1016/j.bbcan.2009.11.002
203. Luke JJ, Onderdonk BE, Bhave SR, Karrison T, Lemons JM, Chang P, et al. Improved survival associated with local tumor response following multisite radiotherapy and pembrolizumab: Secondary analysis of a phase I trialimmunoradiotherapy response in advanced solid malignancies. Clin Cancer Res (2020) 26(24):6437–44. doi: 10.1158/1078-0432.CCR-20-1790
204. Xiao A, Luke JJ, Partouche J, Karrison T, Chmura SJ, Al-Hallaq HA. Evaluation of dose distribution to organs-at-Risk in a prospective phase 1 trial of pembrolizumab and multisite stereotactic body radiation therapy (Sbrt). Pract Radiat Oncol (2022) 12(1):68–77. doi: 10.1016/j.prro.2021.09.005
205. Markovsky E, Budhu S, Samstein RM, Li H, Russell J, Zhang Z, et al. An antitumor immune response is evoked by partial-volume single-dose radiation in 2 murine models. Int J Radiat Oncol Biol Phys (2019) 103(3):697–708. doi: 10.1016/j.ijrobp.2018.10.009
206. Yasmin-Karim S, Ziberi B, Wirtz J, Bih N, Moreau M, Guthier R, et al. Boosting the abscopal effect using immunogenic biomaterials with varying radiation therapy field sizes. Int J Radiat Oncol Biol Phys (2022) 112(2):475–86. doi: 10.1016/j.ijrobp.2021.09.010
207. Lemons J, Luke J, Janisch L, Hseu R, Melotek J, Chmura S. The adscopal effect? control of partially irradiated versus completely irradiated tumors on a prospective trial of pembrolizumab and sbrt per nrg-Br001. Int J Radiat Oncology Biology Phys (2017) 99(2):S87. doi: 10.1016/j.ijrobp.2017.06.209
208. Schneider T, Fernandez-Palomo C, Bertho A, Fazzari J, Iturri L, Martin OA, et al. Combining flash and spatially fractionated radiation therapy: The best of both worlds. Radiotherapy Oncol (2022) 175:169–77. doi: 10.1016/j.radonc.2022.08.004
209. Griffin RJ, Ahmed MM, Amendola B, Belyakov O, Bentzen SM, Butterworth KT, et al. Understanding high-dose, ultra-high dose rate, and spatially fractionated radiation therapy. Int J Radiat Oncol Biol Phys (2020) 107(4):766–78. doi: 10.1016/j.ijrobp.2020.03.028
210. Prezado Y. Divide and conquer: Spatially fractionated radiation therapy. Expert Rev Mol Med (2022) 24:e3. doi: 10.1017/erm.2021.34
211. Yan W, Khan MK, Wu X, Simone CB, Fan J, Gressen E, et al. Spatially fractionated radiation therapy: History, present and the future. Clin Trans Radiat Oncol (2020) 20:30–8. doi: 10.1016/j.ctro.2019.10.004
212. Johnsrud AJ, Jenkins SV, Jamshidi-Parsian A, Quick CM, Galhardo EP, Dings RP, et al. Evidence for early stage anti-tumor immunity elicited by spatially fractionated radiotherapy-immunotherapy combinations. Radiat Res (2020) 194(6):688–97. doi: 10.1667/RADE-20-00065.1
213. Wei J, Montalvo-Ortiz W, Yu L, Krasco A, Ebstein S, Cortez C, et al. Sequence of Apd-1 relative to local tumor irradiation determines the induction of abscopal antitumor immune responses. Sci Immunol (2021) 6(58):eabg0117. doi: 10.1126/sciimmunol.abg0117
214. Dovedi SJ, Adlard AL, Lipowska-Bhalla G, McKenna C, Jones S, Cheadle EJ, et al. Acquired resistance to fractionated radiotherapy can be overcome by concurrent pd-L1 blockade. Cancer Res (2014) 74(19):5458–68. doi: 10.1158/0008-5472.CAN-14-1258
215. Dovedi S, Illidge T. The antitumor immune response generated by fractionated radiation therapy may be limited by tumor cell adaptive resistance and can be circumvented by pd-L1 blockade. Oncoimmunology (2015) 4(7):e1016709. doi: 10.1080/2162402X.2015.1016709
216. Bestvina CM, Pointer KB, Karrison T, Al-Hallaq H, Hoffman PC, Jelinek MJ, et al. A phase 1 trial of concurrent or sequential ipilimumab, nivolumab, and stereotactic body radiotherapy in patients with stage iv nsclc study. J Thorac Oncol (2022) 17(1):130–40. doi: 10.1016/j.jtho.2021.08.019
217. Sundahl N, Vandekerkhove G, Decaestecker K, Meireson A, De Visschere P, Fonteyne V, et al. Randomized phase 1 trial of pembrolizumab with sequential versus concomitant stereotactic body radiotherapy in metastatic urothelial carcinoma. Eur Urol (2019) 75(5):707–11. doi: 10.1016/j.eururo.2019.01.009
Keywords: cancer, metastatic tumor, high-dose radiotherapy, low-dose radiotherapy, immunotherapy
Citation: Ji X, Jiang W, Wang J, Zhou B, Ding W, Liu S, Huang H, Chen G and Sun X (2023) Application of individualized multimodal radiotherapy combined with immunotherapy in metastatic tumors. Front. Immunol. 13:1106644. doi: 10.3389/fimmu.2022.1106644
Received: 24 November 2022; Accepted: 22 December 2022;
Published: 12 January 2023.
Edited by:
Kuangyu Shi, University of Bern, SwitzerlandReviewed by:
Ran Zhu, Soochow University, ChinaXiaotao Zhang, The Affiliated Qingdao Central Hospital of Qingdao University, China
Copyright © 2023 Ji, Jiang, Wang, Zhou, Ding, Liu, Huang, Chen and Sun. This is an open-access article distributed under the terms of the Creative Commons Attribution License (CC BY). The use, distribution or reproduction in other forums is permitted, provided the original author(s) and the copyright owner(s) are credited and that the original publication in this journal is cited, in accordance with accepted academic practice. No use, distribution or reproduction is permitted which does not comply with these terms.
*Correspondence: Xiangdong Sun, sunxd_81@126.com
†These authors have contributed equally to this work